Exogenous source
Origin
Cigarette smoking
Cigarette smoke
Air pollution (ozone, SO2, NO2)
Polluted air
Infection
Bacteria/virus/fungus/protozoa
Endogenous source
Location
Electron transport chain of mitochondria
Endothelial cells
Xanthine oxidase system
Airway epithelial cells
Inflammatory cells
1. NADPH oxidase (NOX)
Phagocytes (neutrophils, macrophages)
2. Oxidative bursts:
Myeloperoxidase (MPO)
Neutrophils, macrophages
Eosinophil peroxidase
Eosinophils, macrophages
Heme peroxidase
Macrophages
3. Nitric oxide synthase (NOS)
5.2.1 Cigarette Smoking and COPD
Cigarette smoking is the most important cause of COPD and is among the leading causes of preventable morbidity and mortality. The most effective way to prevent disease due to cigarette smoking is to prevent smoking initiation. For those who have started smoking, cessation substantially reduces many adverse health consequences. Unfortunately, cessation does not eliminate health consequences of cigarette smoke exposure. Lung cancer risk, for example, remains high for the remainder of an ex-smoker’s life. In COPD, cessation does not result in the restoration of lung function. If cessation occurs early in the course of disease, however, the rate at which lung function is lost with aging normalizes. In the face of more substantial disease, emphysema may progress despite cessation. Understanding the mechanisms that lead to progressive disease despite smoking cessation will be key in preventing smoke-induced diseases for former smokers.
5.2.2 Cigarette Smoke and Oxidative Stress
The most common source of exogenous oxidants is cigarette smoke. Cigarette smoke contains approximately 1015–1017 oxidants and free radicals per puff in the gas phase. Numerous oxidant compounds are present among the 4000–7000 constituents in cigarette smoke. In the particular fraction, phenols and semiquinones are identified among these compounds, while superoxide (O2 −), epoxides, peroxides, nitric oxide (NO), nitrogen dioxide, peroxynitrite, peroxynitrates, and acrolein are included in the gas phase. Cigarette smoke can induce increased oxidant burden and cause irreversible changes to endogenous antioxidant protection in the airways. In addition, the oxidants derived from cigarette smoke damage airway epithelial cells, inducing direct injury to membrane lipids, proteins, carbohydrates, and DNA, which leads to the persistent inflammation that characterizes COPD. The combination of oxidative stress and persistent inflammation accounts for the increased free radicals in the airways and further enhances pro-inflammatory gene expression, inflammatory protein release, and inactivation of antiproteases, leading to a vicious cycle of oxidative injury and inflammatory cell recruitment (Fig. 5.1) [2].


Fig. 5.1
Schema of oxidative stress in COPD pathogenesis
5.2.3 Endogenous Oxidant Generation
The ROS of endogenous origin are produced through enzymatic and nonenzymatic reactions of electron transfer. The principal cellular sites and processes that generate oxidants are the mitochondria, the microsomes, the xanthine/xanthine oxidase system, and the nicotinamide adenine dinucleotide phosphate hydrogen (NADPH) oxidase. The mitochondria are currently recognized to be the main source of endogenous intracellular oxidants through an electron leak from the mitochondrial respiratory chain. The important role of mitochondrial proteolytic enzymes was shown to provide resistance toward oxidative stress [3]. A recent study further highlighted the precious role of mitochondria in COPD pathogenesis: mitochondrial dysfunction in patients with COPD is associated with excessive mitochondrial ROS levels, which contributes to enhanced inflammation and cell hyper-proliferation [4]. In the airways, the endogenous sources of oxidants are alveolar macrophages, epithelial cells, endothelial cells, and recruited inflammatory cells such as neutrophils, eosinophils, monocytes, and lymphocytes. The number of macrophages and neutrophils in cigarette smokers are increased, both in the lung and systemically, generating ROS. The activation of these cells results in the formation of O2 −, which is converted into hydrogen peroxide (H2O2) by the enzyme superoxide dismutase (SOD). Phagocytes from cigarette smokers spontaneously release increased amounts of O2 − and H2O2 compared to those from nonsmokers. H2O2 is also increased in bronchoalveolar lavage fluid (BALF) and exhaled breath condensate (EBC) collected from cigarette smokers [5]. In contrast, the enzymes catalase and glutathione (GSH) peroxidase, which breakdown H2O2, are downregulated in smokers.
5.2.4 Measurement of Oxidative Stress
Oxidative stress markers indicate the level of free oxidants and ROS-generating capacity. However, quantifying them remains difficult because oxidants and ROS are highly reactive. Multiple sample types, such as exhaled air, EBC, sputum, blood, endobronchial or surgical biopsy samples, and BALF, have been used to determine oxidative stress. These materials differ considerably in availability and significance.
H2O2 is a reactive oxygen species that can produce highly reactive oxidants and free radicals. It is also a product of many redox-active metal catalyzed reactions, leading to more oxidant-generating potential. There is some evidence that the concentration of H2O2 is elevated in the EBC and sputum from COPD patients. Determination of EBC pH is a reproducible method of sampling airway acidity; however, it is an indirect and nonspecific marker of oxidative stress. A study has shown that pH in EBC is inversely correlated with respiratory disease conditions such as asthma, COPD, and acute exacerbations [6]. Nitric oxide (NO) and reactive nitrogen species contribute to oxidative injury via their interactions with oxygen radicals. The levels of NO in exhaled air are known to be correlated with the disease activity of bronchial asthma; however, the involvement of NO and reactive nitrogen species in the development of COPD remains controversial. 8-Isoprostane, which is an end product of lipid peroxidation, is relatively stable at physiological temperature and significantly elevated in the EBC from patients with COPD [7]. F2α-Isoprostane, 4-hydroxy-2-nonenal (4-HNE), and malondialdehyde (MDA) are also highly reactive lipid peroxidation products and have been evaluated in samples from COPD subjects. In the case of MDA, it is not clear whether thiobarbituric acid reactive substances (TBARS) or specifically MDA have been measured. MDA/TBARS can be determined in EBC, serum, and biopsy samples. Additionally, 3-nitrotyrosine, 8-hydroxy-deoxyguanosine (8-OH-dG), and protein carbonyls have been investigated to assess oxidative stress in inflammatory lung diseases including COPD. Despite numerous studies aimed at identifying ideal oxidative stress markers, none have been found to be specific for COPD. Therefore, there are as yet no relevant standardized clinical methods or treatment guidelines toward oxidative stress in COPD.
5.3 Oxidative Stress in COPD
Oxidative stress resulting from oxidant and antioxidant imbalance is proposed as the mechanical basis of COPD. Alveolar macrophages and peripheral blood neutrophils from COPD patients are more activated and release increased amount of ROS in the form of the superoxide radical and H2O2. The significance of oxidative stress has been described by several studies that have identified the elevated markers of oxidative stress and free radicals in patients with COPD. The level of 8-OH-dG was increased in urine from COPD patients, and the level of 3-nitrotyrosine and F2α-isoprostane was elevated in the lungs of patients with COPD [8]. Furthermore, these markers demonstrated a strong correlation with COPD severity, defined by forced expiratory volume in the first second (FEV1). The levels of lipid peroxidation products, such as 4-HNE and MDA, were also increased in the lungs and respiratory muscles, and this increase was negatively correlated with lung function [9].
5.3.1 Protein Carbonylation and COPD
Protein carbonylation is a type of protein oxidation that can be promoted by reactive oxygen species. It usually refers to a process that forms reactive ketones or aldehydes that can be reacted with 2,4-dinitrophenylhydrazine (DNPH) to form hydrazones. The development of the antibody against DNPH-derivatized proteins revolutionized the studies of carbonylated proteins by allowing for the use of immunological techniques and, more recently, mass spectrometry. Barreiro et al. reported that protein carbonyls are elevated in the respiratory muscle from severe COPD patients compared with control subjects and further showed that the increase of protein carbonyls was correlated negatively with FEV1 [10]. The same group also reported that the intensity of creatine kinase carbonylation was significantly greater in the quadriceps femoris muscles of patients with COPD as compared with control subjects and correlated negatively with FEV1 and maximal oxygen consumption (VO2 max) [11]. Burcham et al. demonstrated that intermediate filament proteins are targets of acrolein-induced protein carbonylation in A549 cells, providing evidence for the involvement of carbonylation of these proteins during smoke-induced lung injury [12]. Sato et al. showed that protein carbonyls in the lungs of mice tended to increase with aging and were enhanced after chronic exposure of cigarette smoke [13]. Protein carbonylation can modify protein function, disrupting normal cell function and physiological mechanisms.
5.3.2 Oxidative Stress and COPD Pathogenesis
The pathogenesis of COPD involves several pathogenetic processes, such as oxidative stress, inflammation, protease/antiprotease imbalance, apoptosis, and cellular senescence, which are modified by genetic and epigenetic factors (Fig. 5.1). In particular, oxidative stress has several important consequences for the pathogenesis of COPD.
Oxidative stress activates the transcription factor nuclear factor-kB (NF-kB) pathway, leading to an increase in inflammation. NF-kB expression and activation are increased in the lungs from COPD subjects and correlate with airflow limitation. ROS are also intracellular second messengers, acting as inflammatory stimuli that induce micro-oxidative bursts, which are essential for cellular activation. The ability of corticosteroids to repress pro-inflammatory gene expression is impaired in COPD because of oxidative stress. Carbonylation and nitration reduce the activity and expression of histone deacetylase 2 (HDAC2), which is essential for the suppression of activated inflammatory genes and the anti-inflammatory effects of corticosteroids. HDAC2 is markedly reduced in COPD since there is another pathway activated by oxidative stress, phosphoinositide-3-kinase-δ, which is responsible for reducing HDAC2 activity and expression [14, 15]. Nuclear factor erythroid 2-related factor 2 (Nrf2) is a key transcriptional factor that regulates a number of antioxidant and cytoprotective genes. Loss of HDAC2 activity in COPD has also been demonstrated to lead to loss of Nrf2 activity through increased Nrf2 acetylation, thereby decreasing Nrf2 stability and expression [16]. Another transcriptional corepressor, sirtuin 1 (SIRT1), is similarly impacted by oxidative stress, reducing both its expression and activity leading to an accelerated aging process and the development of emphysema. Many studies have analyzed the relationship between aging and oxidative stress. Moderate oxidative stress may gradually develop with age because plasma levels of lipoperoxidation products and antioxidant enzyme activities in red blood cells increase with aging, whereas plasma levels of nutritional antioxidants decrease. Senescence marker protein-30 (SMP30), a gluconolactonase involved in L-ascorbic acid biosynthesis that is known to decrease with aging in rats and mice, has been shown to protect mouse lungs from oxidative stress associated with aging and smoking [13]. Oxidative stress can result in enhanced inflammatory gene expression, failure to resolve the inflammatory response, corticosteroid insensitivity, impaired antioxidant defenses, and accelerated aging, all of which are associated with the development of COPD pathology.
Oxidative stress can potentiate the effect of proteases on COPD through the activation of enzymes such as serine proteases, cysteine proteases, and matrix metalloproteinases. ROS increase the activity of matrix metalloproteinases by activating metalloproteinase precursors. The increase of epithelial and endothelial cell apoptosis in the lungs has been known to result in tissue destruction and in the development of emphysema. There are several reports that demonstrated the association between oxidative stress and apoptosis. Vascular endothelial growth factor (VEGF) is a pluripotent growth factor that has a broad impact on endothelial cell survival and its function and plays a critical role in the maintenance of lung structure. An experimental emphysema in rat lungs induced by VEGF blockade demonstrated that apoptosis predominates in the lungs under oxidative stress and that the blockade of apoptosis decreased the expression of oxidative stress markers [17]. The administration of the SOD mimetic can also prevent the development of alveolar cell apoptosis. Petrache et al. demonstrated that SOD overexpression protects against alveolar cell apoptosis and alveolar enlargement induced by ceramide, a second messenger lipid, which is upregulated in the lungs with smoking-induced emphysema [18, 19]. Koike et al. also reported that the administration of vitamin C to SMP30 knockout mice following chronic exposure of cigarette smoke attenuates oxidative stress and alveolar septal cell apoptosis, restoring the concentration of VEGF in the BALF and in the lungs [20].
5.4 Antioxidants in the Lung
Antioxidants are agents that decrease steady-state ROS and protect cellular macromolecules from oxidative modification. Antioxidants can be classified into two categories: enzymatic and nonenzymatic. The principal components of the enzymatic antioxidants are SOD, catalase, glutathione (GSH) peroxidases, GSH S-transferase, peroxiredoxin, and the heme oxygenase (HO). Nonenzymatic antioxidants include GSH, uric acid, bilirubin, ferritin, vitamin A (retinol), vitamin C (ascorbic acid), vitamin E (α-tocopherol), carotenoids, and polyphenols.
5.4.1 Superoxide Dismutases and COPD
SODs are a class of enzymatic antioxidants that catalyze the dismutation of superoxide into oxygen and hydrogen peroxide. There are three forms of superoxide dismutase in humans: Cu/Zn SOD, MnSOD, and extracellular SOD (EcSOD). Cu/Zn SOD is the most abundant in the lung tissue and is especially concentrated in ciliated epithelial cells. MnSOD is predominantly located in airway wall cells and mitochondria of the lungs. EcSOD is the major SOD in the extracellular fluid that exists in abundance in the lungs. The levels of EcSOD were increased in sputum from smokers, whereas the activity of EcSOD was reduced in COPD patients, and polymorphism in the EcSOD gene has been linked to both reduced lung function in COPD and susceptibility to emphysema [21].
5.4.2 Glutathione Redox System and COPD
GSH is a vital intracellular and extracellular protective antioxidant because of its strong electron-donor potential via the sulfhydryl groups of cysteine residues and its high concentration in cells. GSH is more concentrated in the epithelial lining fluids and plays an important role in the maintenance of the redox balance in the lungs. Glutathione peroxidases catalyze a variety of GSH reactions including the breakdown of H2O2. The levels of GSH are upregulated in the BALF from chronic smokers. However, this increase in GSH levels might not be enough to neutralize the excessive load of oxidants during acute exposure to cigarette smoke, since a depletion of GSH is observed after chronic cigarette smoke exposure in a time and dose-dependent manner.
5.4.3 Catalase, Heme Oxygenase, and COPD
Catalase is a common enzymatic antioxidant found in living organisms, which catalyzes the decomposition of H2O2 to water and oxygen. Betsuyaku et al. demonstrated that catalase is significantly downregulated in pulmonary macrophages from COPD patients compared with those that have never smoked and ex-smokers without COPD [22].
HO is an enzyme that catalyzes the degradation of heme and generates bilirubin, iron, and carbon monoxide. These downstream products of heme catabolism mediate the antioxidant properties of HO. HO-1 expression is upregulated in several respiratory diseases including COPD. Shinohara et al. demonstrated that overexpression of HO-1 in the lungs of mice attenuates elastase-induced pulmonary emphysema [23]. Bilirubin is one of the HO-1 downstream products and has been known to have potent antioxidant properties. A recent study showed that higher serum bilirubin concentration is associated with a higher FEV1 and less annual decline of FEV1 in patients with mild to moderate COPD. This suggested that bilirubin has a protective effect on COPD disease progression and a potential to be an easy access biomarker for this disease [24]. More recently, an in vivo study further indicated that the administration of bilirubin attenuated smoking-induced pulmonary injury by suppressing inflammatory cell recruitment and pro-inflammatory cytokine secretion, increasing anti-inflammatory cytokine levels and SOD activity [25].
5.4.4 Dietary Antioxidants and COPD
Dietary antioxidants include the micronutrients vitamins, carotenoids, and polyphenols. Vitamins A, C, and E and carotenoids (including lycopene which is a major carotenoid in tomatoes) have been well studied, possess strong antioxidant activities, and are well absorbed with relatively high bioavailability. As they are unable to be synthesized in humans, their concentration in serum/plasma, as well as a local milieu in the lungs, is reflected by dietary intake of vegetables and fruits as major sources. Several epidemiologic studies showed the association between lung function and dietary intake of vitamins and carotenoids, as well as manipulating antioxidant-rich diets, has led to altered clinical outcomes in chronic respiratory diseases including COPD [26]. Many studies have reported reduced levels of antioxidant nutrients, such as vitamins A, C, and E and carotenoids in serum or plasma from COPD patients. Recently, Kodama et al. showed that plasma vitamin C, lycopene, and total carotenoid levels were significantly lower in Japanese COPD patients than that of healthy elderly people [27]. Furthermore, Ford et al. demonstrated the prospective associations between concentrations of antioxidant nutrients and all causes of mortality among adults with obstructive lung function in the United States, showing that only the concentrations of vitamin C and lycopene were found to be significantly and inversely associated with all causes of mortality [28]. Interestingly, animal studies, which showed the benefits of both vitamin C and lycopene supplementation to COPD model mice, have already been reported. As noted above, vitamin C treatment successfully prevented cigarette smoke-induced pulmonary emphysema in SMP30 knockout mice, a strain that is genetically engineered to preclude vitamin C synthesis [20]. Similarly, lycopene also prevented cigarette smoke-induced emphysema in the senescence-accelerated mouse prone 1 by decreasing oxidative stress in the lungs [29]. Overall, the intake of these antioxidant nutrients could be beneficial for the treatment of COPD by modulating oxidative stress in this disease. However, it has so far failed to meet expectations in several clinical trials.
On the other hand, the polyphenols are a family of complex molecules that are ubiquitous in plants and include the flavonoids and phenolic acids. Dietary intake of polyphenols has been reported to improve the symptoms, FEV1, and arterial oxygen tension in COPD patients. Resveratrol, a polyphenolic compound derived from red wine, was able to improve both corticosteroid efficacy and lung function in a general population. Resveratrol significantly reduced steroid-resistant cytokines from bacterial endotoxin-exposed alveolar macrophages from COPD patients [30]. Similarly, curcumin, a phenolic yellowish pigment and ingredient of turmeric, has been found to modulate cigarette smoke- or peroxide-induced steroid resistance by restoring HDAC2 activity and expression [31]. Suzuki et al. has also demonstrated that oral curcumin administration significantly attenuates elastase- and cigarette smoke-induced pulmonary emphysema in mice [32]. However, the efficacy of many polyphenols is limited either by low bioavailability or by transformation in the gastrointestinal tract.
Although there is not enough evidence to indicate the direct relationship between vitamin D and oxidative stress in COPD, vitamin D supplementation may be beneficial because the majority of COPD patients have vitamin D deficiency [33]. Many in vitro studies demonstrated important anti-inflammatory and immunomodulatory effects of vitamin D. A recent in vivo study showed that vitamin D deficiency both accelerates lung function decline and aggravates the development of emphysema following chronic exposure to cigarette smoke [34]. Moreover, a recent systematic review and meta-analysis reported that major deficiencies in 25-hydroxyvitamin D were associated with COPD severity [35]. Collectively, these studies indicate that vitamin D supplementation may be an important therapeutic strategy, in particular, for the prevention of COPD exacerbation, which is worthy of further investigation.
5.5 Genetic and Epigenetic Regulation of Oxidative Stress in COPD
Although cigarette smoking is clearly the most common risk factor in the development of COPD, only a small portion of smokers develop the disease, highlighting the important role of the individual’s genetic predisposition to COPD. In addition, due to the heterogeneity of this disorder, epigenetic regulation of specific molecular and cellular pathways is also believed to be relevant to COPD pathogenesis. Epigenetics describes heritable changes in gene expression that do not involve changes to the underlying DNA sequence. Epigenetic change is a regular and natural occurrence but can also be influenced by several factors including age, environment, and disease state. DNA methylation, histone modification, and noncoding RNA-associated gene silencing are currently considered to initiate and sustain epigenetic changes.
5.5.1 Genetic Predisposition in COPD
The alpha 1-antitrypsin gene, SERPINA1, is the best described genetic risk factor for COPD; the most frequent mutation causing severe alpha 1-antitrypsin deficiency among Caucasians is called PI*Z. However, many genes are supposed to contribute to the individual predisposition to this multifactorial disorder. Major COPD susceptibility loci have recently been identified on chromosome 4 by genome-wide association studies [36]. The hedgehog-interacting protein (HHIP), a developmental transcription factor, was identified as a COPD susceptibility gene, while family with sequence similarity 13 member A (FAM13A) was associated with the regulation of oxidative stress and lung function. On chromosome 15, nicotinic acetylcholine receptor (CHRNA3/5) loci were associated with increased smoking intensity and emphysema in COPD patients. On chromosome 2q, SERPINE2 and XRCC5 have been linked to lung function and airflow obstruction in family-based genetics studies. Several genetic mutations in antioxidant genes are identified: polymorphisms in glutathione S-transferase (GST), superoxide dismutase 3 (SOD3), and microsomal epoxide hydrolase (EPHX1) are associated with the rapid decline of lung function characteristic of COPD [37]. Apart from the inherited genetic changes, the acquired somatic mutations constitute an important component in the pathogenesis of COPD. The oxidative stress in COPD can directly damage lung DNA. The distribution of oxidative damage in the genome depends on the varying susceptibilities of sequences to oxidative attack and preferential targeting of repair processes.
5.5.2 DNA Methylation and Histone Acetylation in COPD
DNA methylation is shown to regulate the expression of pro-inflammatory genes during the development of COPD. In patients with COPD, DNA methylation of the promoters of pro-inflammatory genes has been observed both in airway epithelial cells and in alveolar macrophages. Furthermore, methylation of the p16 gene promoter was frequently observed in the sputum of patients with COPD and positively correlated with heavy cigarette smoking. A recent analysis of methylation and gene expression indicated that DNA methylation is a genome-wide phenomenon in small airways of patients with COPD, and associated with altered expression of genes and pathways important to COPD, such as the Nrf2 oxidative response pathway [38].
The histone modifications include acetylation, methylation, ubiquitination, phosphorylation, and sumoylation. In particular, histone acetylation may play a role in airway diseases through the involvement of HDAC in inflammation. Indeed, HDAC2 is an important corepressor protein in inflammation. It is suggested that oxidants and free radicals could alter this sensitive protein, causing reduced expression level of these deacetylases in the lungs of smokers and COPD patients [14]. Furthermore, HDAC2 downregulation impairs Nrf2 activation in the lung by decreasing the half-life of Nrf2 [39]. SIRT1 is a class III HDAC with anti-inflammatory, anti-senescence, and anti-apoptotic activity mediated by the deacetylation of histone and nonhistone proteins, including transcription factors such as NF-kB and forkhead box O3 (FOXO3). It has been reported that SIRT1 is reduced in the lungs of cigarette smoke-exposed mice and patients with COPD and that SIRT1 attenuates cigarette smoke-induced lung inflammation and injury. A recent animal study showed that SIRT1 protects against cigarette smoke-induced oxidative stress, which is mediated by FOXO3 but is independent of Nrf2 [40].
5.5.3 MicroRNA Regulation upon Cigarette Smoking and in COPD
MicroRNAs (miRNAs) are noncoding endogenous RNAs, approximately 19–25 nucleotides in length, which play a role in the posttranscriptional regulation of gene expression. miRNA biogenesis begins with the cleavage of a pri-miRNA into a pre-miRNA, which is mediated by the Drosha enzyme (Fig. 5.2). The pre-miRNA is then actively transported from the nucleus into the cytoplasm by exportin-5. Next, the RNase III enzyme Dicer processes the pre-miRNA to generate a double-stranded RNA. One strand is degraded and the other is incorporated into the RNA-induced silencing complex (RISC), which then functions as a mature miRNA against its target mRNAs. The mature miRNAs, which are expressed during development in a tissue- or cell type-specific manner, mediate posttranscriptional repression of gene expression by increasing mRNA degradation or by inhibiting translation. This is achieved by direct binding between the miRNA 5′ region and the 3′ untranslated region of the target mRNA. miRNAs may also indirectly alter gene expression by epigenetic mechanisms such as DNA methylation and histone acetylation. These processes enable a fine-tuning of gene expression as opposed to an on-off switch.


Fig. 5.2
MicroRNA biogenesis and oxidative stress
miRNAs have been associated with diverse biological processes such as the development, cellular differentiation, and pathogenesis of various diseases including COPD (Table 5.2). Graff et al. found that cigarette smoking downregulates global miRNA expression in human alveolar macrophages, possibly by modification of Dicer [41]. Similarly, Izzotti et al. reported a downregulation of miRNA expression in the lungs of cigarette smoke-exposed rats [42]. Schembri et al. examined whole-genome miRNA and mRNA expression in the bronchial airway epithelium in current smokers versus those who have never smoked and found that miR-218 levels modulate airway epithelial gene expression in response to exposure to cigarette smoke [43]. In contrast, Ezzie et al. compared the miRNA profile in the lung tissue from non-COPD smokers with that in the lung tissue from COPD subjects and found that 70 miRNAs are differentially expressed (57 miRNAs are upregulated and 13 miRNAs downregulated) and that miR-15b, in particular, is upregulated in COPD [44]. miR-15b was increased in COPD samples compared with controls and was differentially expressed in correlation with disease severity by the Global Initiative for Chronic Obstructive Lung Disease classification. The authors have further described that expression of Smad7, which is a validated target for miR-15b, is decreased in bronchial epithelial cells in COPD. More recently, Van Pottelberge et al. analyzed the miRNA profile from induced sputum samples and showed that let-7c is significantly reduced in COPD subjects compared with smokers without COPD [45]. Hassan et al. examined the effect of cigarette smoke extract (CSE) on human bronchial epithelial cells and showed that miR-101 and miR-144 are upregulated by CSE and suppress the cystic fibrosis transmembrane conductance regulator protein, a chloride channel involved in the maintenance of fluid homeostasis in the lung [46].
Table 5.2
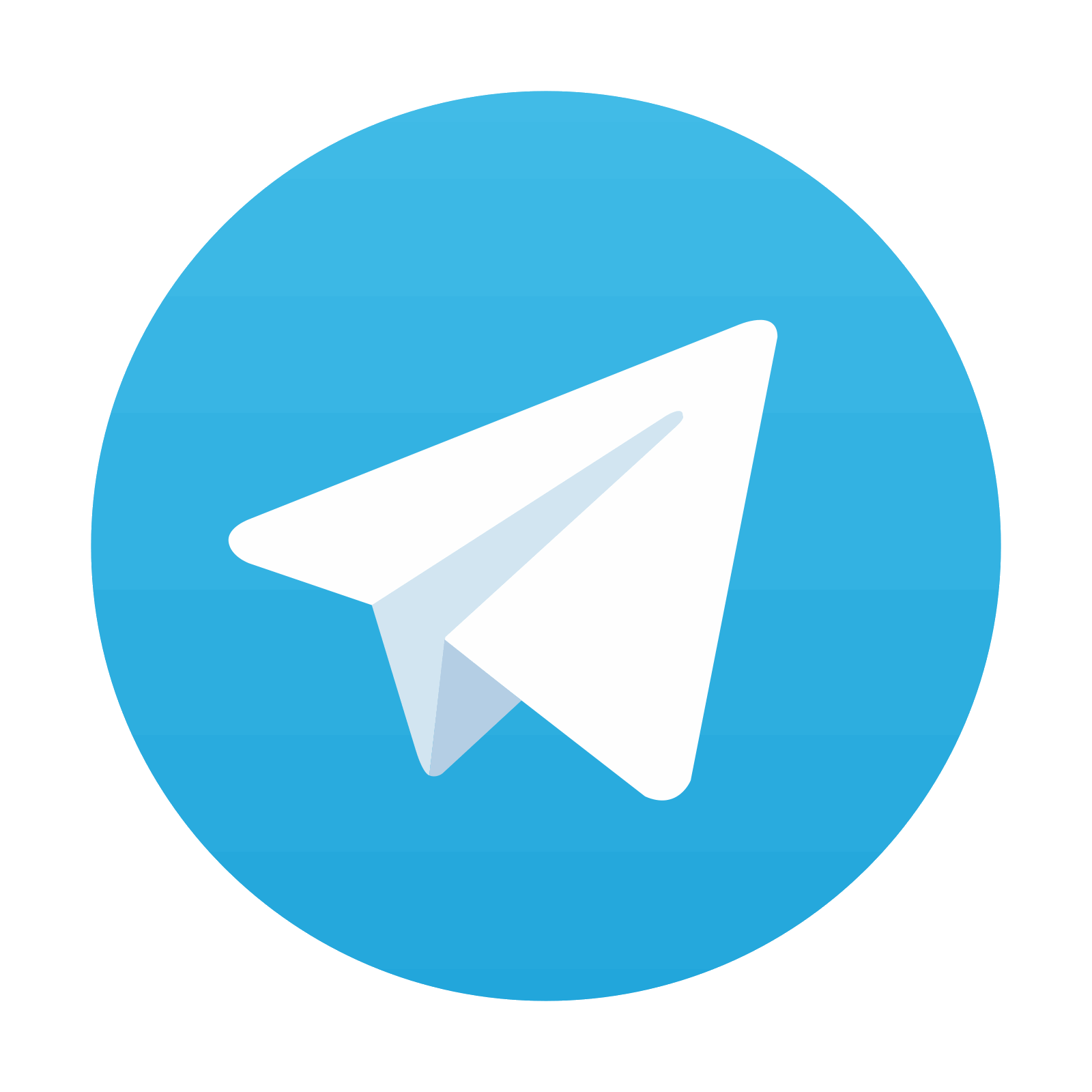
Representative microRNAs associated with smoking and COPD
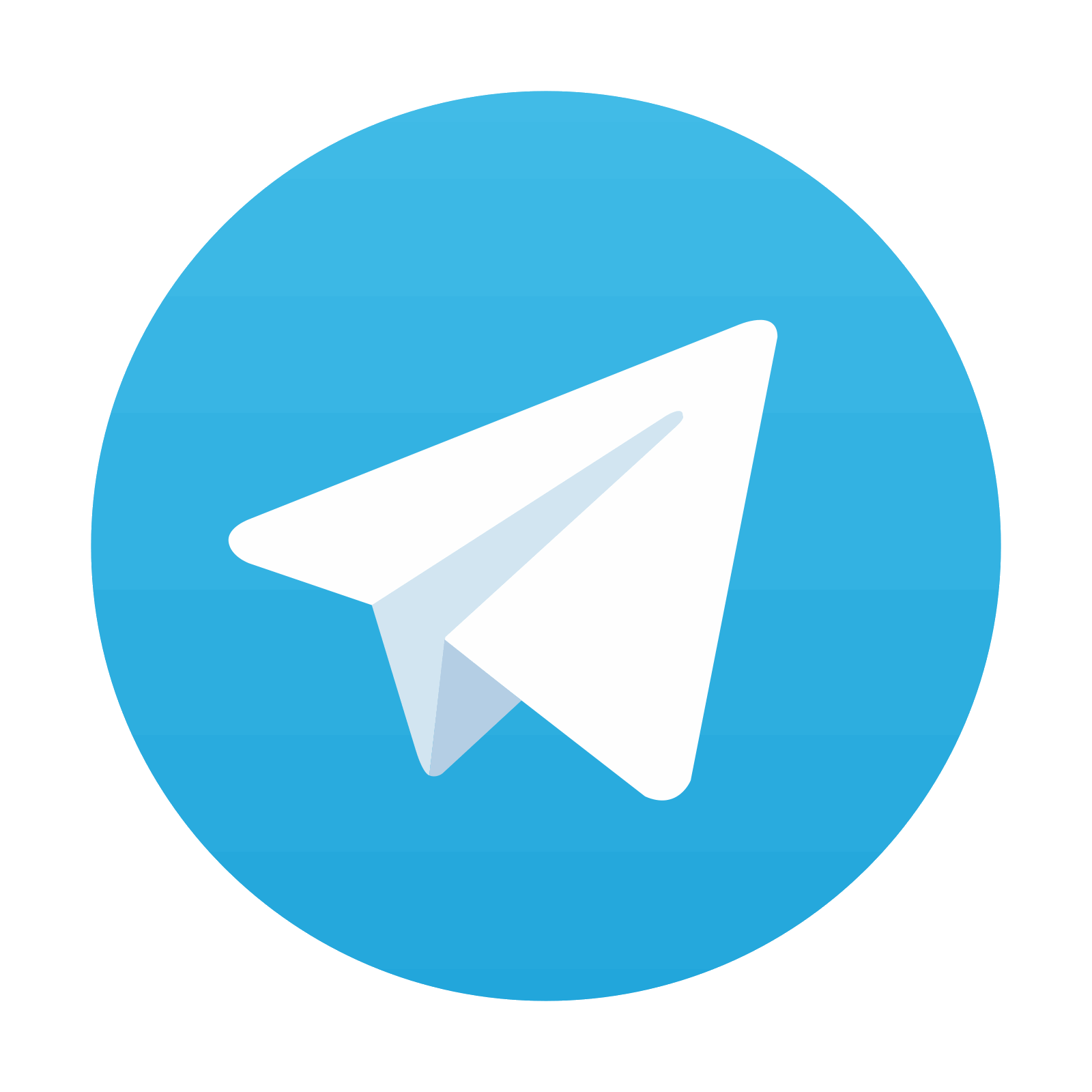
Stay updated, free articles. Join our Telegram channel
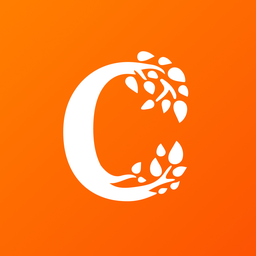
Full access? Get Clinical Tree
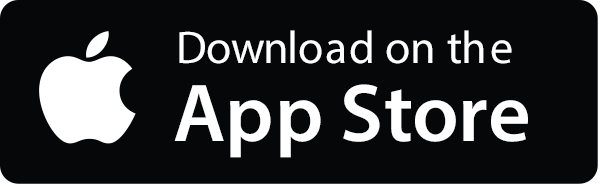
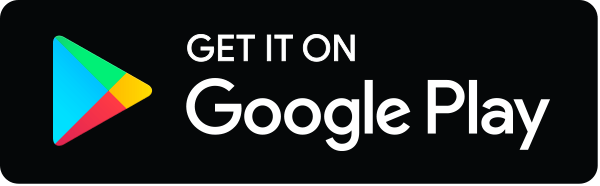
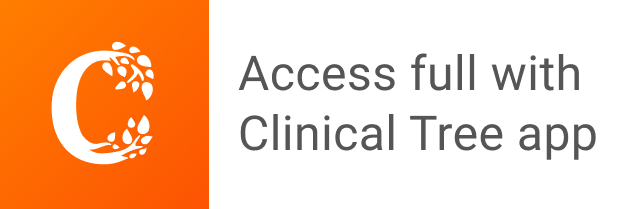