(1)
Project-team INRIA-UPMC-CNRS REO Laboratoire Jacques-Louis Lions, CNRS UMR 7598, Université Pierre et Marie Curie, Place Jussieu 4, Paris Cedex 05, France
Abstract
Other important signaling mediators include: (1) endogenous, gaseous, diffusible messengers, or gasotransmitters, such as carbon monoxide (CO; Sect. 10.2), nitric oxide (NO; Sect. 10.3), and hydrogen sulfide (H2S; Sect. 10.4), in addition to oxygen and carbon dioxide, as well as several reactive oxygen (ROS) and nitrogen species (RNS; Sect. 10.6), that act as intra- and intercellular regulators; (2) membrane-bound enzymatic complexes such as the reduced form of nicotinamide adenine dinucleotide phosphate oxidase (NADPH oxidase or NOx; Sect. 10.5); (3) some coregulators, such as (protein kinase) A-kinase-anchoring proteins (AKAP; Sect. 10.8.4) and annexins (Sect. 10.8.5); and (4) transcription factors involved in stress response, such as the proteic complex nuclear factor κ light-chain-enhancer of activated B cells (NFκB; Sect. 10.9.1), the heterodimer hypoxia-inducible factors (HIF; Sect. 10.9.2), and members of the Forkhead box (Fox; Sect. 10.9.3) and P53 families (Sect. 10.9.4; Table 10.1).
Other important signaling mediators include: (1) endogenous, gaseous, diffusible messengers, or gasotransmitters, such as carbon monoxide (CO; Sect. 10.2), nitric oxide (NO; Sect. 10.3), and hydrogen sulfide (H2S; Sect. 10.4), in addition to oxygen and carbon dioxide, as well as several reactive oxygen (ROS) and nitrogen species (RNS; Sect. 10.6), that act as intra- and intercellular regulators; (2) membrane-bound enzymatic complexes such as the reduced form of nicotinamide adenine dinucleotide phosphate oxidase (NADPH oxidase or NOx; Sect. 10.5); (3) some coregulators, such as (protein kinase) A-kinase-anchoring proteins (AKAP; Sect. 10.8.4) and annexins (Sect. 10.8.5); and (4) transcription factors 1 involved in stress response, such as the proteic complex nuclear factor κ light-chain-enhancer of activated B cells (NFκB; Sect. 10.9.1), the heterodimer hypoxia-inducible factors (HIF; Sect. 10.9.2), and members of the Forkhead box (Fox; Sect. 10.9.3) and P53 families (Sect. 10.9.4; Table 10.1).
Table 10.1
Classification of some transcription factors based on their function and mode of activation (Sources: [1131, 1132]; bHLH: basic helix–loop–helix; bZip: basic leucine zipper; AP1: Activating protein-1 (Fos and Jun components); ATF: activating transcription factor; β-Ctn: β-catenin; C/EBP: bZip CCAAT [(cytidine)2–(adenosine)2–thymidine) box motif]/enhancer-binding protein; CREB: cAMP response element-binding protein; DREAM: Downstream regulatory element antagonistic modulator; ER: estrogen receptor; ETS: E twenty-six; Fox: Forkhead box; GATA: DNA GATA sequence-binding transcription factor; GR: glucocorticoid receptor; GTF: general transcription factor; HIF: hypoxia-inducible factor; HNF: hepatocyte nuclear factor: MeCP2: Methyl-CpG-binding protein-2 transcriptional repressor; MEF: myocyte enhancer factor; MRF: bHLH myogenic regulatory factor; MyF: myogenic factor [MRF set]; MyoD: myocyte differentiation transcription factor [MRF set]; NF1: nuclear factor-1; NFAT: nuclear factor of activated T cells; NFκB: nuclear factor-κB; ONR: orphan nuclear receptor; PiT1: pituitary-specific positive transcription factor-1, or POU domain-containing class-1 transcription factor 1 [POU1F1]; PPAR: peroxisome-proliferator-activated receptor; RAR: retinoic acid receptor; RXR: retinoid X receptor; SMAD: small (son of, similar to) mothers against decapentaplegia homolog; SP1: Specificity protein-1; SREBP: sterol regulatory element-binding protein; SRF: serum response factor: STAT: signal transducer and activator of transcription; TR: thyroid hormone receptor; TuLP: Tubby-like protein).
Constitu- | Conditionally active | |||||
---|---|---|---|---|---|---|
tively | transcription factors | |||||
active, | Develop- | Signal-dependent | ||||
nuclear | mentally | Nuclear | Internal | Cell-surface | ||
transcrip- | regulated | factors | signal- | receptor-dependent | ||
tion | regulated | Resident | Shuttle | Latent | ||
factors | nuclear | cyto- | ||||
plasmic | ||||||
C/EBP | GATA | ER | ATF6 | AP1 | DREAM | βCtn |
GTF2 | HNF | GR | HIF | ATMs | FoxO | Gli |
NF1 | Hox | PR | P53 | CREB | MITF | |
SP1 | MyF1/5/6 | TR | SREBP | E2F1 | NFAT | |
MyoD (MyF3) | PPAR | ONRs | ETS | NFκB | ||
PiT1 | RAR | MeCP2 | SMAD | |||
Winged-helix | RXR | MEF2 | STAT | |||
(FoxA-FoxQ) | MyC | TuLP | ||||
SRF | NotchICD |
More than 2000 transcription factors are encoded in the human genome. Some transcription factors reside permanently in the nucleus and are constitutively active (functional class 1). Regulatory, conditionally-active transcription factors (class 2) constitute 2 sets: developmentally regulated transcription factors (class 2.A), often cell type-specific, and signal-dependent transcription factors (class 2.B) that are subdivided into extracellular ligand-dependent nuclear receptors, or nuclear factors (class 2.B.1), among which some are activated by certain types of hormones; internal signal-regulated (class 2.B.2); and cell-surface receptor-regulated (class 2.B.3) transcription factors. The latter category is split into nuclear resident (class 2.B.3.a), shuttle (between the nucleus and cytoplasm; class 2.B.3.b ), and latent cytoplasmic (class 2.B.3.c) transcription factors that translocate into the nucleus upon receptor activation.
10.1 Gaseous Neurotransmitters
Different categories of chemical species constitute a set of 50 to 100 neuro- and gliotransmitters, neuromodulators, and cotransmitters, in addition to acetylcholine and adenosine [1133]: (1) biogenic amines (e.g., adrenaline, noradrenaline, dopamine, histamine, serotonin, and tryptamine); (2) amino acids (aspartate, glutamate, and glycine, as well as Daspartate and Dserine, which is released by protoplasmic [grey matter] type-2 astrocytes close to NMDA-type glutamate receptor synapses), in addition to GABA synthesized by glutamic acid decarboxylase; (3) peptides (enkephalins, substance-P, and other neuropeptides, especially those of neurons of the digestive tract, such as vasoactive intestinal polypeptide, cholecystokinin, gastrin, and insulin, the enteric nervous system using nearly all transmitters of the central nervous system); (4) gases (CO, NO, and H2S).
Most often, neurotransmitters are stored in nerve terminals and signal via both fast and slow mechanisms, using ionotropic and metabotropic receptors, respectively. Signaling is terminated by specific reuptake carriers and/or degradation.
Nitric oxide functions as a neuromodulator and/or -transmitter in the central, peripheral, and enteric nervous systems. In the enteric nervous system, NO is produced in enteric neurons by NOS1 subtype. It acts as an inhibitory neurotransmitter. It diffuses from neurons to adjacent smooth muscle cells, in which it activates soluble guanylate cyclase, thereby relaxating smooth muscle cells.
In cardiac nodal cells, nitric oxide supports acetylcholine release to augment vagal neurotransmission and trigger bradycardia [1134]. Isoform NOS1 is detected in parasympathetic ganglion that innervates the sinoatrial node. Nitric oxide acts presynaptically to facilitate vagal neurotransmission via the cGMP–PDE3 pathway that promotes cAMP–PKA-dependent phosphorylation of presynapticCaV2.2 channels to increase presynaptic calcium influx and acetylcholine release. In addition, NO can function postsynaptically via a cGMP-dependent increase in the hyperpolarization-activated current in sinoatrial cells. Moreover, NO can be generated upon M2 muscarinic receptor stimulation of NOS3 and preclude the adrenergic stimulation of CaV1.2 channels via the PDE2–cAMP axis [1134].
Once glutamate is bound to NMDA-type receptor ion channel, calcium enters the neuron, binds calmodulin to activate NOS1 synthase. Freely diffusible NO enters adjacent neurons or other cell types, links to iron in heme of soluble guanylate cyclase at the postsynaptic membrane near NMDA-type glutamate receptors to form cGMP messenger [1133].
Hydrogen sulfide (H2S) acts on serotoninergic neurons in the dorsal raphe nucleus [1133].
Like other gasotransmitters, CO is neither stored in synaptic vesicles nor released by exocytosis, and do not act at postsynaptic membrane receptors. In specific neurons of the brain such as olfactory neurons, CO is synthesized by constitutive heme oxygenase (HO2) and can activate soluble guanylate cyclase. Carbon monoxide is also produced by heme oxygenase in collaboration with cytochrome-P450 reductase and biliverdin reductase. Heme oxygenase has inducible (HO1) and constitutive (HO2) isoforms. Heme oxygenase-1 is involved in cellular responses to stress. Heme oxygenase-2 resides in neurons of the brain, enteric neurons, and interstitial pacemaker cells of Cajal in the the gastrointestinal tract. Interstitial cells of Cajal produce low NOS1 levels. Interstitial cells of Cajal, enteric neurons, and intestinal smooth muscle cells interact to cuase smooth muscle relaxation.
Nitric oxide and carbon monoxide operate as coneurotransmitters produced by the same neurons not only in the brain, but also in the enteric nervous system. Their respective synthases, neuronal NO synthase (NOS1) and heme oxygenase-2 (HO2), colocalize in enteric neurons [1135]. In small intestinal (jejunal and ileal) smooth muscle cells, NO signaling requires CO generation for muscle relaxation and inhibitory non-adrenergic non-cholinergic neurotransmission that controls intestinal motility.
10.2 Carbon Monoxide and Heme Oxygenases
Carbon monoxide (CO), a stable, lipid-soluble gas, is produced by heme oxygenase (HO), the rate-limiting enzyme of the catabolism of heme [1136].2 Although CO can bind to heme-containing proteins, or hemeproteins, (but not vesicles) for transient storage and later release,3 the predominant source is de novo synthesis [1137]. It then diffuses according to the local partial pressure gradient. Carbon monoxide binds to the ferrous iron of heme and, then, influences the activity of all hemeproteins.
10.2.1 Carbon Monoxide Synthesis and its Regulation
Two CO sources — HO-dependent (major) and HO-independent — exist. The latter results from oxidation of organic molecules, phenols, and flavenoids and peroxidation of lipids during severe stress [1136].
Heme oxygenase catalyzes heme degradation to carbon monoxide, iron, and biliverdin with the oxidation of reducing agents NADH and NADPH.4 Heme oxygenase is responsible for the recycling of iron from senescent and damaged erythrocytes and other cell types, such as hepatocytes. Heme oxygenase allows to limit the overall production of reactive oxygen species, as excess free heme catalyzes the formation of these species. Among other agents, atrial natriretic peptide and donors of nitric oxide are important modulators of the heme–heme oxygenase couple [1138].
Heme oxygenase has 2 active membrane-bound isoforms encoded by 2 distinct genes: inducible HO1 and constitutive HO2 subtypes. Therefore, HO2 participates in the immediate response and HO1 in long-term regulation.5 Isozyme HO1 acts as a heat shock and stress protein induced by several agents that cause oxidative damage. Induction of HO1 coupled with ferritin synthesis yields a rapid, protective, anti-oxidant response. In skin fibroblasts, it protects against UV-induced oxidative stress [1136]. Heme oxygenases are anchored to the endoplasmic reticulum membrane. They also localize to the nuclear envelope and perinuclear region.
10.2.1.1 Heme Oxygenase-1
Heme oxygenase-1 is expressed basally only in the liver and spleen, but can be produced in most tissues. It participates in the degradation of erythrocytes and elimination of toxic heme.
In pulmonary artery endothelial cells, HO1 resides in plasma membrane caveolae. Caveolin can bind to HO1 and regulate its activity.
Oxidative stress upregulates HO1 in various tissues.6 Cell-specific inducers of HO1 includes heme, cobalt protoporphyrin, hemin (oxidized heme),7 ROS and RNS (e.g., peroxynitrite), transition metal complexes (iron FeCl2 and FeCl3 and cobalt CoCl2), NO, and oxidized lipids. Unlike in other cell types, in cerebral vascular endothelial cells, hydrogen peroxide, arachidonic acid, NO donors (3-morpholinosydnonimine and Snitroso Nacetyl dl-penicillamine), transition metals, TNFα, and glutamate fail to upregulate HO1 enzyme.
Acute and chronic productions of HO1 reduces concentrations of vasoconstrictors such as 20HETE. In particular, HO1 impedes the activity of heme-containing thromboxane synthase and cyclooxygenase-2 [1136]. Chronic, modest expression of HO1 increases adiponectin, hence, resistance to oxidative stress.
10.2.1.2 Heme Oxygenase-2
Heme oxygenase-2 has its highest expression in the central nervous system and testes. Consequently, activity of heme oxygenase in the brain exceeds that in other organs. In the brain, HO2 is highly produced in vascular endothelial and smooth muscle cells as well as perivascular astrocytes.8 Carbon monoxide is also synthesized in neurons. In the brain, HO2 associates with guanylate cyclase, ALA synthase, cytochrome-P450 reductase, and nitric oxide synthase [1136]. In addition, HO2 concentration is relatively high in keratinocytes, but low in human dermal fibroblasts.
Like other gaseous mediators, CO causes a vasodilation of cerebral arterioles. Certain vasodilatory stimuli, such as hypoxia, reduced arterial pressure in the autoregulation range, and ADP, raise CO concentration owing to a rapid increase in HO2 activity, but not HO2 synthesis. Only glucocorticoid hormones augment HO2 production, as a glucocorticoid response element exist in the Ho2 gene promoter [1137]. Glucocorticoids are slow-acting, weak inducers of HO2 enzyme.
Elevated heme concentration, Ca
influx, and Ca
–calmodulin-dependent processes also elevate HO2 activity [1137]. Post-translational phosphorylation (activation) of HO2 indeed depends on Ca
–calmodulin. On the other hand, bilirubin may yield a negative feedback on HO2 function. In hippocampal and olfactory neurons, heme oxygenase-2 is activated by casein kinase-2 and protein kinase-C.



In endothelial cells, HO2 is stimulated by protein Tyr kinases as effectors of the Glu–iGluR axis. In fact, CO synthesized by HO2 in astrocytes is responsible for glutamate-induced dilation of pial arterioles. The CO production in endothelial cells and astrocytes rises in response not only to glutamate, but also tumor-necrosis factor-α.
Brain production of CO causes an accumulation in the cerebrospinal fluid, especially in the cortical, periarachnoid space (basal CO concentration 50–80 nmol).
10.2.2 Vasodilation and Other Effects
Carbon monoxide not only causes vasodilation, but also supports action of vasodilators and attenuates that of vasoconstrictors such as platelet-activating factor [1137]. Its vasorelaxant effect results not only from a cGMP-dependent, but also cGMP-independent, stimulation of some K + channels and an increase in adiponectin (NO-dependent vasodilation) [1136] (Table 10.2).
Table 10.2
Effects of carbon monoxide and other products of heme oxygenase activity (Source: [1136]; ⊕ : stimulation; ⊖ : inhibition ↑: increase; ↓: decrease; ND: not determined; AP1: activator protein-1; BK: high-conductance, Ca
-activated, voltage-gated K + channel; CO: carbon monoxide; COx: cyclooxygenase; CyP: cytochrome-P450; GSH: anti-oxidant glutathione (reduced form); HETE: hydroxyeicosatetraenoic acid; HO: heme oxygenase; MAPK: mitogen-activated protein kinase; MnSOD: manganese-containing superoxide dismutase; PKC: protein kinase-C; ROS: reactive oxygen species; RSK, ribosomal S6 kinase; sGC: soluble guanylate cyclase; SOD ec : extracellular superoxide dismutase; TxaS1: thromboxane-A synthase-1 [or CyP5a1]). Uncoupling of nitric oxide synthase (NOS) can result from glucose that enhances superoxide (O2 − ) production. The latter promotes the formation of peroxynitrite (ONOO − ), which oxidizes NOS cofactor tetrahydrobiopterin, thereby uncoupling NOS, i.e., favoring O2 − production instead of NO synthesis. Prostaglandin-A is a potent inducer of HO synthesis to protect against stress and hypoxia, particularly in cardiomyocytes. Hypoxia fosters the production of HO in vascular smooth muscle cells but limits that of endothelin-1, platelet-derived growth factor-B, and vascular endothelial growth factor in endothelial cells.

Target | Effect |
---|---|
BK channel | Vasodilation |
SOD ec | ↑ |
GSH | ⊕ , especially in endothelial cells |
20HETE | ⊖ (hence, vasodilation) |
P38MAPK | ⊖ cell proliferation, |
⊖ inflammation, apoptosis | |
MnSOD | Anti-oxidant |
RSK | ⊖ apoptosis |
sGC | cGMP synthesis, vasodilation |
ND | Angiogenesis |
Heme–HO system | |
HO | ⊖ CyP (TxaS1), COx (heme degradation, |
resulting vasodilation) | |
Biliverdin | ↓oxidative and nitrosative stress |
Bilirubin | ROS scavenger, |
inhibition of NADPH oxidase and PKC, | |
anti-inflammatory effect | |
Biliverdin reductase | AP1 complex activation |
Carbon monoxide relaxes vascular smooth muscle cells viaBK channels. It indeed binds to BK channel and increases Ca
sparks that activate BK channels. In the arterial wall, the transient BK current provokes a membrane hyperpolarization that reduces the activity of voltage-dependent Ca
channels. Both CO and CO-releasing molecule CORM3 stimulate BK channels [1136].


Both NO and CO activate BK channels on vascular smooth muscle cells, but targeting different channel subunits. Heme possesses a high affinity for the heme-binding domain (Cys-Lys-Ala-Cys-His) of the α subunit of BK channel. Binding of heme to the heme-binding domain inhibits the BK channel. Carbon monoxide tethers to channel-bound reduced heme, thereby relieving heme-induced inhibition of the BK channel and leading to channel activation [1137]. In addition, CO binding increases Ca
sensitivity of the BK channel. Furthermore, HO enzyme and BK channel colocalize; HO1 and HO2 can reduce inhibition caused by heme, as they destroy heme.

On the abluminal side of the neurovascular unit, glutamate binds to ionotropic (iGluR) and metabotropic (mGluR) glutamate receptors of astrocytes, causing a Ca
influx and increasing HO2 activity. On the luminal side, endothelial nitric oxide and prostacyclin contribute to the response. The former activates guanylate cyclase, thereby producing cGMP messenger. The latter stimulates the PGI2 receptor (IP) that triggers the ACase–cAMP pathway. Carbon monoxide is much less efficient in activating guanylate cyclase than nitric oxide. Nevertheless, cGMP acts as a mediator of CO-induced vasodilation. Activated protein kinase-G can phosphorylate ryanodin receptors of the sarcoplasmic reticulum, thereby increasing Ca
spark frequency and BK channel activity.


In the carotid body, HO2 colocalizes with BK channels. Carbon monoxide generated by HO2 may mediate O2 sensitiviy of BK channels in glomus cells of the carotid body [1137]. Carbon monoxide may also serve as a neurotransmitter in the carotid body [1136]. However, HO2 is not necessary for O2 sensing by cells of the carotid body and adrenal medulla.
During hypoxia, CO reduces the proliferation of endothelial cells, as it impedes VEGF production by adjacent vascular smooth muscle cells, as well as that of vascular smooth muscle cells via cGMP [1137]. Cytosolic messenger cGMP activates P38MAPK, thus upregulating caveolin-1 that prevents cell proliferation. In a different context, CO can promote the proliferation of microvascular endothelial cells.
Heme oxygenase-1 and its product CO participate in the maintenance of the cell homeostasis, including DNA stability and repair to ensure cell survival [1139]. DNA repair pathways encompass repair of single- and double-strand DNA breaks (Vol. 1 – Chaps. 4. Cell Structure and Function and 5. Protein Synthesis). Double-strand DNA breaks are detected initially by damage sensors that trigger the recruitment to altered DNA of proper kinases and their activation.9 The HO1–CO axis contributes to DNA repair of double-strand DNA breaks via ATMK kinase.
Endogenous CO at nanomolar levels primes the production of anti-oxidant enzymes, such as heme oxygenase-1 and mitochondrial superoxide dismutase SOD2, to protect against oxidative stress [1140]. Hence, pro-oxidant and cytotoxic CO primes a protective response.
Gaseous signaling molecules carbon monoxide and nitric oxide also stimulates mitochondrial genesis via cytochrome-C oxidase. In the heart, carbon monoxide works via transcriptional activation of peroxisome proliferator-activated receptor-γ coactivator PGC1α, nuclear respiratory factor-1 and -2, and mitochondrial transcription factor-A (TFaM) [1140].
10.2.3 Carbon Monoxide and Nitric Oxide
Endothelial nitric oxide and prostacyclin cooperate with the HO–CO axis to regulate the blood circulation. Nitric oxide heightens HO2 activity in cerebral microvessels using a cGMP-dependent mechanism. In aortic endothelial cells, NO stimulates the CO production. However, CO inhibits NO-mediated relaxation when the NO concentration rises. On the other hand, CO stimulates soluble guanylate cyclase and cGMP when the NO concentration falls [1136]. Vasodilatory effect of CO thus depends on BK channel activity as well as NO concentration and soluble guanylate cyclase.
In addition, in cerebral arterioles, whereas acute production of CO causes a vasodilation, prolonged exposure of cerebral arterioles to elevated CO produces progressive constriction, as it inhibits nitric oxide synthase [1137]. Moreover, NO can hinder HO2 activity.
The HO–CO axis protects the vasculature. Carbon monoxide precludes apoptosis and generation of endogenous oxidants [1137].10 Carbon monoxide binds to a heme prosthetic group and regulates components of cell signaling, such as BK channels and guanylate cyclase. It inhibits NADPH oxidase and cytochrome-C oxidase of the mitochondrial respiratory chain, thereby hampering ROS formation. In addition, bilirubin is a potent scavenger of reactive oxygen species.
Whereas NO can simulate cystathionine γ-lyase, this enzyme can be inhibited by CO [1137]. Gasotransmitter H2S can inhibit HO2 in aortic endothelial cells.
10.3 Nitric Oxide, Nitric Oxide Synthases, Nitrite, and Nitrate
Nitric oxide (NO) a highly diffusible gas that runs very rapidly from its site of synthesis and crosses quickly cell membranes. Nitric oxide can act as an intra- and autocrine second messenger as well as a paracrine regulator on neighboring cells.
However, NO is a labile vasodilator with a limited half-life (
[1 s]); its effects are thus localized close to the site of release. This free radical is highly reactive with other chemical species, notably oxygen, superoxide, and iron-containing hemes that act as NO scavengers.

This stable radical possesses an unpaired electron (hence the notation NO ∙ ).11 Extracellular nitric oxide was first designated as endothelium-derived relaxing factor, as this messenger is involved in the regulation of vascular tone and blood flow (Vol. 5 – Chap. 9. Endothelium).12
The primary source for NO synthesis in the blood circulation is endothelial nitric oxide synthase. Nitric oxide is generated from the enzymatic oxidation of Larginine by nitric oxide synthase. In particular, synthesized endothelial NO contributes to the control of the vasomotor tone and, hence, blood pressure. However, NO can also be produced from the reduction of nitrite and nitrate. Inorganic nitrate derives either from NO oxidation or from diet. It can serve as a storage form of reactive nitrogen oxides that can be reduced back to nitrite and nitric oxide. Release of NO from stored nitrite and nitrate compensate the very short half-life of NO [1141].
Nitric oxide can complex with all transition metals, the so-called metal nitrosyl complexes (MNO), or simply metal nitrosyls (e.g., Cu
, Mn
, and Fe3 + ).13 Nitric oxide interacts with metalloproteins, especially those that contain hemes, and with thiols. Nitric oxide can act in the form of free radical (NO or NO ∙ ) or as chemical species, such as nitrosyl (NO + ) or nitroxyl (NO − ) ions or Snitrosothiols (SNO). Hemes either transform NO into nitrate or promote its transformation into Snitrosothiols.


Nitric oxide controls: (1) at the tissue level, neurotransmission and vascular tone; (2) at the cell level, gene transcription (as it binds to iron-responsive elements) and mRNA translation; and (3) at the molecule level, post-translational modifications of proteins (e.g., adpribosylation).
10.3.1 Nitric Oxide Synthases
Nitric oxide is synthesized by nitric oxide synthases (NOS). Three nitric oxide synthases (NOS1–NOS3) differs according to subcellular location, gene expression, protein interactions, post-translational modifications, and catalytic behavior for specific roles. Yet, these different isoforms share a similar synthesis mode that uses Larginine and molecular oxygen (O2), reduced nicotinamide adenine dinucleotide phosphate (NADPH) as substrates and flavin adenine dinucleotide (FAD), flavin mononucleotide (FMN), and (5,6,7,8)-tetrahydrobiopterin (BH4) as cofactors to form nitric oxide. All NOS isoforms bind to calmodulin. They contain heme. Moreover, these widespread isozymes can coexist in many cell types. In particular, NO is continuously synthesized and released from the vascular endothelium.
Nitric oxide synthases require oxygen and other cofactors, especially tetrahydrobiopterin and Ca
–calmodulin for efficient production of nitric oxide.14 Both NADPH and O2 serve as cosubstrates. In addition to pteridine tetrahydrobiopterin, NO synthesis also relies on other cofactors, such as flavin mononucleotide and adenine dinucleotide, and reduced thiols. Synthesis of NO depends on substrate and oxygen availability, hence on blood supply. It is altered by endogenous NOS inhibitor asymmetric dimethylarginine.

In the absence of pteridine tetrahydrobiopterin, NOS3 dimer is uncoupled from Larginine oxidation and oxygen is reduced to form superoxide anion (O2 − ) instead of nitric oxide.
Active nitric oxide synthases are homodimers, whatever the isoform. Enzymes NOS are hemeproteins; heme irons can bind NO in both ferric or ferrous states within seconds from the start of NO synthesis. Nitric oxide binding to heme reversibly inhibits NOS catalysis (negative feedback by the inactive NOS–NO complex). Displacement of NOS away from the cell cortex can decrease its activity.
Nitric oxide synthases transfer electrons from reduced NADPH to FAD and FMN in the C-terminal reductase domain to heme in the N-terminal oxygenase domain. On the other hand, NOS has a limited capacity to reduce O2 to superoxide. The oxygenase domain also binds BH4 cofactor, O2, and Larginine [1142]. At the heme site, electrons are used to reduce O2 and oxidize Larginine, thereby producing Lcitrulline and nitric oxide.15
All NOS isoforms bind calmodulin. Both NOS1 and NOS3 connect to calmodulin upon intracellular Ca
influx (half-maximal activity 200–400 nmol) [1142]. Calmodulin facilitates the electron transfer from NADPH in the reductase domain to the heme in the oxygenase domain. Calmodulin links to NOS2 at very low intracellular Ca
concentrations ( ∼ 40 nmol), i.e., with high affinity even in the absence of Ca
ion, because of a different amino acid structure of the calmodulin-binding site [1142].



All NOS proteins contain a zinc–thiolate cluster formed by a zinc ion with 2 CXXXXC (CysXXXXCys) motifs (one motif from each monomer) at the NOS dimer interface [1142]. Zinc has a structural rather than catalytic function. This site actually tethers to BH4 and Larginine.
Electron transfer from the reductase domain enables NOS ferric (Fe3 + ) heme to bind O2 and form a ferrous (Fe
)–dioxy species. This species may receive a second electron from BH4 or the reductase domain [1142]. Oxidized BH4 is the trihydrobiopterin radical (BH3 ∙ ) or trihydropterin radical cation (protonated at N5; BH3
). Trihydrobiopterin radical or radical cation can be recycled to BH4 by NOS. Alternatively, reducing agents such as ascorbic acid (AscH; concentration
[1 mmol] in cells) can reduce BH3 ∙ back to BH4, hence producing ascorbate radical (Asc ∙ ) [1142].



10.3.1.1 Constitutive NOS1 and NOS3
Two Ca
-dependent constitutive NOS isoforms include neuronal NOS (nNOS or NOS1) and endothelial NOS (eNOS or NOS3).16 Calcium-activated calmodulin binds to and transiently activates constitutive NOS dimers.

Transiently activated constitutive NOS synthesizes NO in response to: (1) increased intracellular calcium concentration by different activators followed by calmodulin binding and (2) other stimuli such as fluid flow stress exerted at the wetted surface and within the vassel wall.
NOS1
Nitric oxide synthase-1 is, in particular, located in synapses of central (brain and spinal cord) and peripheral (sympathetic ganglia, adrenal glands, and nitrergic nerves) neurons. It resides also in other cell types such as skeletal muscle cells, in which an alternatively spliced variant exist. It is detected in epithelial, kidney macula densa, pancreatic islet, and vascular smooth muscle cells.
Nitric oxide synthase-1 operates in synaptic remodeling in the central nervous system, relaxation of corpus cavernosum and penile erection, central regulation of blood pressure, and smooth muscle relaxation, especially vasodilatation primed by peripheral nitrergic nerves, as NOS1-derived NO acts as a neurotransmitter that stimulates NO-sensitive guanylate cyclase in effector cells [1142]. Neuronal NOS1 is involved in neurogenesis, learning, memory, and long-term regulation of synaptic transmission (long-term potentiation or inhibition).
Enzymes NOS1 and NOS3 have distinct roles in the vascular tone regulation. In particular, NOS1 is implicated in the blood flow rate control in the human forearm and coronary circulation, independently of its effects in the central nervous system [1142]. On the other hand, NOS3 provokes vasodilation in response to acetylcholine, substance-P, or mechanical stress.
Synthase NOS1 contains a PDZ domain used for between-protein interactions that determine NOS subcellular distribution and activity. Isozyme NOS1 interacts [1143]: (1) in the nervous system with anchoring adaptors Disc large homologs DLg2 and DLg4,17 as well as (2) in the striated muscle with α1-syntrophin. Caveolin-3 binds to NOS1 and inhibits NO synthesis.
NOS3
Nitric oxide synthase NOS3 has the weakest activity with respect to NOS1 and NOS2 subtypes. It is expressed not only by vascular endothelia, but also by airway epithelia as well as other cell types, such as neurons, cardiomyocytes, platelets, kidney tubular epithelial cells, and syncytiotrophoblasts of the human placenta).
In endothelial cells, NOS3 synthesizes NO markedly when intracellular Ca
rises. Calcium ion provokes the binding of calmodulin to NOS3 enzyme. Several other proteins also interact with NOS3 and regulate its activity. Heat shock protein HSP90 links to NOS3 and serves as an allosteric activator that promotes NOS3 recoupling [1142].

In endothelial cells, NOS3 generates NO in response to mechanical stress as well as acetylcholine and bradykinin. Nitric oxide released from endothelial cells diffuses in the neighborhood to regulate smooth muscle cell tone and proliferation, platelet aggregation, and leucocyte adhesion to the endothelium. Endothelial NOS-derived NO is a vasodilator and an inhibitor of platelet aggregation and adhesion to the vascular wall as well as smooth muscle cell proliferation (as it precludes PDGF secretion) and production of matrix molecules and leucocyte adhesion to vascular endothelium and subsequent diapedesis (as it impedes the production of many cell adhesion molecules).
Endothelial NOS also intervenes in lung morphogenesis and postnatal angiogenesis [1142]. Enzyme NOS3 produced by bone marrow stromal cells supports via NO the mobilization of endothelial progenitor cells by VEGF factor, hence neovascularization.
Post-translational processing (primarily by acylation) incorporates NOS3 to plasmalemmal caveolae. This compartmentation facilitates between-protein interactions and signal transduction. Within caveolae, NOS3 is targeted by G-protein-coupled receptors as well as other receptors (estrogen receptor and high-density lipoprotein receptor ScaRb1) [1144]. Binding to caveolin-1 inhibits NO synthesis by NOS3 [1145].
Enzyme NOS3 can be myristoylated, palmitoylated, farnesylated, acetylated, and phosphorylated. These modifications assist in recruiting the enzyme to various cell compartments. Except phosphorylation, these changes do not significantly affect NOS3 activity.
Nitric oxide synthase NOS3 can generate both nitric oxide and superoxide.18 In the presence of Ca
–calmodulin, NOS3 produces NO from LArg by means of electron transfer from NADPH via a flavin-containing reductase domain to oxygen-bound at the heme of an oxygenase domain, which also contains binding sites for tetrahydrobiopterin and LArg [1146]. In the absence of tetrahydrobiopterin (BH4), NO synthesis is abrogated and instead superoxide is generated. The NOS3 uncoupling caused by S-glutathiolation may be triggered by other uncoupling processes such as BH4 depletion and may further enhance BH4 depletion.

Thiols potentiate NOS3 activity. Subtype NOS3 possesses specific redox-sensitive thiols. These thiols can be S-glutathiolated.19 This oxidative modification switches NOS3 from a NO synthase function to an NADPH-dependent oxidase generation of O2 − . Under oxidative stress, S-glutathiolation occurs via a thiol–disulfide exchange with oxidized glutathione or reaction of oxidant-induced protein thiyl radicals with reduced glutathione. S-glutathiolation of NOS3 reversibly decreases NO production and increases in O2 − generation.20 In endothelial cells, S-glutathiolation of NOS3 is associated with an impaired endothelium-dependent vasodilation [1146].
Overexpressed cardiac NOS3 increases concentrations of nitrite, nitrate, and nitrosothiol not only in the heart, but also plasma and liver, and ensures cytoprotection of remote organs after blood transport of nitrite and Snitrosothiol [1147].
Protein kinases PKA, PKB, and AMPK phosphorylate (Ser1177; activate) NOS3 enzyme. On the other hand, PKC phosphorylates (Thr495; inactivate)21 NOS3 as well as promotes dephosphorylation (Ser1177), hence inhibiting NOS3 [1148] (Tables 10.3 and 10.4).22 Phosphatases PP1 and PP2 dephosphorylate NOS3 (Thr495 and Ser1177, respectively).
Table 10.3
NOS3 phosphorylation (Phosphoryl.) and dephosphorylation (Dephosphoryl.). Ser1177 phosphorylation leads to NOS3 activation, and Thr495 phosphorylation to NOS3 inactivation (Source: [1148]).
Ser1177 | Thr495 | |||
---|---|---|---|---|
Phosphoryl. | Dephosphoryl. | Phosphoryl. | Dephosphoryl. | |
(activation) | (inhibition) | (inhibition) | (activation) | |
Phosphatases | PP2 | PP1 | ||
Kinases | PKA | PKC | PKC | PKA |
PKB | (via PP2) | AMPK | (via PP1) | |
PKG | ||||
AMPK | ||||
CamK2 |
Table 10.4
Stimulators of multisite NOS3 phosphorylation and dephosphorylation of specific serine and threonine residues (Source: [1149]).
Residue | Phosphorylation | Dephosphorylation |
---|---|---|
site | stimulators | stimulators |
Ser114 | Mechanical stress, | ATP, |
HDL | VEGF | |
Ser615 | ATP, bradykinin, | |
VEGF | ||
Ser633 | Mechanical stress, | |
ATP, bradykinin, | ||
VEGF | ||
Ser1177 | Mechanical stress, | |
ATP, bradykinin, histamine, | ||
thrombin, hydrogen peroxide, | ||
insulin, estrogen, | ||
adiponectin, leptin, | ||
sphingosine 1-phosphate, | ||
VEGF, IGF1 | ||
Thr495 | Bradykinin, hydrogen peroxide, | |
VEGF |
10.3.1.2 Inducible NOS2
Cytokine-inducible nitric oxide synthase (iNOS or NOS2), or immunocyte NOS,23 produces NO only in selected tissues such as lung epithelium, but in many cell types, such as cardiac, endothelial, vascular smooth muscle, and glial cells, as well as dermal fibroblasts, macrophages, and CD8 + T lymphocytes.
Nitric oxide intervenes in host defense. It is typically synthesized in response to inflammation, i.e., in response to lipopolysaccharide and cytokines, among other agents. It is implicated in vascular diseases and transplant rejection.
The NOS2 activity appears slowly after exposure to cytokines, is sustained, and can function independently of calcium and calmodulin. However, calmodulin can be tightly bound to NOS2 enzyme.24 Cells regulate NOS1 via aggresome [1150]. The dynein–dynactin complex associates NOS2 to the aggresome at the microtubule-organizing center.
Inducible NOS2 in non-stimulated T lymphocytes is implicated in atherosclerosis and graft-induced intimal thickening. The JaK–STAT signaling primed during T-cell activation inhibits NOS2 expression. Endothelial cells and other stromal cells induce NOS2 expression in CD8 + T cells, at a greater extent than in CD4 + T lymphocytes. Inducible NOS2 in resting T cells and low NO concentrations increase T-cell proliferation in response to allogeneic endothelial cells of grafted vessels [1151]. Induction of NOS2 depends on NFκB that is inhibited by STAT factor.
Both soluble and membrane-associated chloride intracellular channel ClIC4 are sensitive to the redox state. This P53- and MyC-responsive pro-apoptotic protein intervenes in innate immunity as well as in PKC-dependent differentiation of keratinocytes [1152]. In keratinocytes, ClIC4 channel also supports TGFβ signaling, as it hinders interaction of SMAD2P and SMAD3P with PPM1a phosphatase [1152]. In macrophages, ClIC4 is encoded by an early response gene, which is a transcriptional target of NFκB and interferon regulatory IRF3 factor [1152]. Its synthesis is hampered by anti-inflammatory glucocorticoid receptor. Many of ClIC4 functions depend on its nuclear localization. Its S-nitrosylation enables connection to nuclear import proteins. Nuclear translocation of ClIC4 channel indeed relies on NOS2 activation in pro-inflammatory peritoneal macrophages [1152]. Nuclear ClIC4 deactivates the pro-inflammatory program in macrophages to allow the phenotype transition from microorganism struggle to tissue repair.
Asymmetric dimethylarginine and monomethyl arginine inhibit all NOS isoforms.25 Asymmetric dimethylarginine is a cardiovascular risk factor because it reduces NO signaling, hence eliciting endothelial dysfunction and augmenting systemic and pulmonary blood pressure [1153]. Its plasmatic concentration rises particularly in pulmonary hypertension.
Short-lived nitric oxide is rapidly oxidized to nitrite in aqueous solutions; It can also be directly oxidized to nitrate in the presence of superoxide or oxyhemoglobin.
10.3.2 Nitric Oxide Production from Nitrite and Nitrate
Additional sources of nitric oxide than nitric oxide synthases arise from the cycling of nitrate, nitrite, and nitric oxide. Several pathways modulate the NO3 − –NO2 − –NO cycling, such as oxidation or reduction by hemoglobin, myoglobin, neuroglobin, xanthine oxidoreductase (XOR), nitric oxide synthase, carbonic anhydrase, cytochrome-C oxidase, cyclooxygenase, microsomal cytochrome-P450, mitochondrial aldehyde oxidase, and cytochrome-C, as well as bacteria, in different tissues under different conditions [1141, 1154]. Hemoglobin operates at normal pH and oxygen saturation in the vasculature.
Free radical gases are actually synthesized by the body’s cells and transported nearby or remotely to influence cell function and signaling. Nitric oxide has a short half-life ( < 2 ms) in blood. Nitric oxide freely diffuses to adjacent cells, but the diffusion distance in tissues is relatively limited. Nitric oxide half-life within normoxic tissues has been estimated less than 0.1 s with a diffusion distance dependent on oxygen concentration, NO being metabolized by heart mainly to nitrite [1204]. Moreover, NO short half-life in blood due to hemoglobin consumption limits remote NO action [1205].
Nevertheless, NO can be transported as nitrite (NO2 − ) and Snitrosothiol. Nitrites can indeed serve as both a stored NO precursor used for intravascular endocrine NO transport, and an oxidation product of NO metabolism [1206]. Plasma contains approximately 7 μmol of Snitrosothiols, of which 96% are Snitrosoproteins (mainly relatively long-lived serum Snitroso-albumin) that have endothelium-derived relaxing factor-like properties [1207]. Nitric oxide activates specific soluble guanylate cyclase (Sect. 11.2). It also acts via cGMP-independent mechanisms.
10.3.2.1 Nitrite
Nitrite (concentration 0.3–1.0 μmol in plasma and 1–20 μmol in tissues) is an oxidative product of nitric oxide and reduction element of nitrate (NO3 − ) by commensal bacteria, in addition to dietary sources. Nitrites represent a major storage form of nitric oxide in blood and tissues, as NO2 − is much more stable than NO or Snitrosothiols.
Nitrite-Mediated Vasodilation
Nitrites (10 μmol–2 mmol) cause vasorelaxation of isolated aortic rings and decrease both systolic and diastolic blood pressure. Nitrite can also prime vasodilation in isolated vessels at therapeutic ( ≤ 200 μmol) and physiological concentrations (100–200 nmol) [1154].
Nitrite concentration may be higher in the erythrocyte ( ∼ 290 nmol) than in the plasma ( ∼ 120 nmol) [1154]. It depends on the rate of nitrite import in and export out of the erythrocyte.
Nitrite forms a storage pool of nitric oxide that can be mobilized to trigger vasodilation during hypoxia, but at relatively large time scale. In fact, the conversion of free NO to nitrate is much faster than NO2 − reduction to nitric oxide.
Hemoglobin (Hb) is an important NO buffer and a modulator of NO bioavailability. Free NO produced in an erythrocyte is very quickly scavenged by both oxy- or deoxyhemoglobin [1154]. Nitrite may cross membranes by simple diffusion coupled with protonation–deprotonation according to concentration gradients. Nitrite transport across the erythrocyte is regulated by O2 content and deoxyHb-mediated nitrite reduction, thus preventing nitrite export from the erythrocyte by inhibiting anion exchanger-1 (or SLC4a1).26
Nitrite anion is reduced to nitric oxide as oxygen concentration decays by several mechanisms that rely on xanthine oxidoreductase,27 deoxyhemoglobin, and deoxymyoglobin, among others. Members of the heme globin family (cyto-, hemo-, neuro-, and myoglobin) can act as nitrite reductases that regulate the hypoxia-induced NO generation [1156].
Hemoglobin-mediated nitrite reduction is much faster under partially oxygenated than fully oxygenated conditions. Nitrite reduction kinetics by erythrocytic or cell-free Hb are directly regulated by oxygen fractional saturation; the bell-shaped relation reaches a maximal nitrite reduction rate around the oxygen partial pressure at which Hb is 50% oxygenated (HbO 2 P50) [1154]. Nitrite reduction thus becomes faster as erythrocytes are deoxygenated. The combination of deoxygenated erythrocytes and nitrite primes the NO-mediated signaling.
Nitrites, which are not vasoactive at physiological concentrations, can be reduced to nitric oxide in particular by hemoglobin [1157]. Hemoglobin acts as a reductase that then releases NO under allosteric control during its transition from the high-oxygen affinity R (relaxed) to low-oxygen affinity T (tense) state [1158].28 When hemoglobin is exposed to NO2 − , ferrous nitrosyl hemoglobin (
HbNO) is formed. Then, free NO can be possibly produced according to the following reaction [1158]:29

NO2 − +
Hb + H + → Fe 3 + Hb + NO + OH − .

Afterward, released NO can rapidly bind to deoxygenated hemoglobin to produce nitrosylhemoglobin (
Hb–NO) or react with any oxygenated hemoglobin chains to form methemoglobin and nitrate [1155]:




Many chemical reactions associate NO, nitrite, and various forms of hemoglobin (oxyHb, deoxyHb, metHb, HbNO, and HbSNO) [1154]. Reaction 1 is the initial step in the deoxyHb–nitrite reaction that may create the transient Fe 3 + Hb intermediate. In the reversible reaction 2, Fe 3 + Hb generates metHb and NO. However, in the erythrocyte, NO unlikely rebinds to metHb. Reaction 3 related to nitrite and oxyHb is characterized by a slow initiation phase and a fast autocatalytic phase. Yet, the autocatalytic phase is unlikely in the erythrocyte because of the competition of intermediate species. Reaction 4 corresponds to the reversible binding of nitrite to metHb.30 Reaction 5 of nitrite-bound metHb (Fe 3 + Hb
) with NO forms reduced deoxyHb and N2O3. The latter may diffuse out of the erythrocyte and subsequently generate NO and nitrite. Alternatively it can react with a thiol (e.g., glutathione or cysteine; reaction 6) followed by export of the nitrosothiol, hence of NO activity. In reaction 7, HbSNO results from the
HbNO intermediate. In reaction 8 (intramolecular transfer), NO + from
Hb
is transferred within Hb to Cysβ93. Alternatively (as in reductive nitrosylation), NO + from
Hb
can also reacts with OH − to generate nitrite.31 In reaction 9, N2O3, a strong nitrosating agent, results from the reaction of nitrite with the Fe 3 + HbNO intermediate. Reaction 10 represents NO binding to deoxyHb. In reaction 11 (oxidative denitrosylation), intermediates in the oxyHb–nitrite reaction oxidize the ferrous nitrosyl heme, thus producing NO and a ferric heme. Reaction 12 deals with the NO dioxygenation reaction that limits the export of NO from the erythrocyte, in which NO reacts with oxyHb to form metHb and nitrate. In addition, nitrite-dependent ATP export out of the erythrocyte can contribute to vasodilation. Hemoglobin that has reacted with nitrite releases membrane-bound glycolytic enzymes, which then produce intracellular ATP for subsequent secretion under hypoxia.






The reduction of nitrite back to NO by deoxyhemoglobin in erythrocytes can be followed by NO transport when its scavenging by hemoglobin is bypassed. Nitric oxide can be transferred to the Cysβ93 thiol group of hemoglobin to form S-nitrosylated hemoglobin when heme nitrosylated hemoglobin (
Hb++NO) is oxygenated [1158]. Subsequent to the formation of Snitrosohemoglobin,32 S-transnitrosylation, the transfer of NO groups to thiols on the erythrocyte membrane and then in the plasma, enables NO delivery to the vascular wall.

Oxyhemoglobin is an efficient NO scavenger. Because nitrite can be reduced to NO by deoxyhemoglobin, an arteriovenous gradient of blood of nitrite exists. Nitrite is consumed between artery (176 ± 10 nmol) and vein (143 ± 7 nmol); this consumption rises during exercise.33
The short lifespan of nitric oxide in blood ( < 2 ms) requires a mechanism to retain NO activity in the circulation, especially in the microcirculation, where the oxygen partial pressure is lower and less oxygen is available for NO synthesis by endothelial nitric oxide synthase. Nitrite can deliver active NO to the vasculature after its reduction by deoxyhemoglobin in erythrocytes.34
10.3.2.2 Nitrate
Both nitrate and nitrite are generated from endogenous and dietary sources. The main dietary source of nitrate is leafy green and root vegetables. The primary endogenous source of nitrate and nitrite is NO that is rapidly oxidized in biological tissues, including blood, into these anions.
Nitric oxide reacts with oxyhemoglobin in erythrocytes to form nitrate and ceruloplasmin and oxygen in plasma to form nitrite. Approximately half of plasma nitrite comes from dietary nitrate and half from oxidation of endogenous NO [1159].
Inorganic nitrate is serially reduced to nitrite and, then, NO, nitrosothiols, and other bioactive nitrogen oxides via numerous pathways in biological tissues, including blood.
Exogenous Nitrate
Ingestion of nitrate amounts from 1 to 2 mmols per day (western diet) [1141]. A diet rich in fruit and vegetables is an important source of nitrate.35 Dietary nitrate is rapidly and completely absorbed in the upper digestive tract. About 60% ingested nitrate is excreted in the urine within 48 h. The half-life of an oral dose of inorganic nitrate is estimated to range from 5 to 8 h, due to reabsorption into the proximal renal tubule. The urinary clearance of nitrate equals about 26 ml/mn.
Endogenous Nitrate
Nitric oxide synthesized by NOS is rapidly oxidized to nitrate in the presence of oxyhemoglobin, which yields approximately 1 mmol nitrate per day [1141]. Methemoglobin is a by-product of this oxidation process that cannot bind oxygen. It needs to be reduced back to hemoglobin by methemoglobin reductase.
Distribution and Excretion
Whatever the source, circulating nitrate is concentrated in the salivary glands and subsequently secreted into the mouth. The salivary nitrate concentration is at least 10 times that of plasma [1141]. Local anaerobic bacteria can reduce nitrate to nitrite. The resulting salivary nitrite concentration is more than 1000 times greater than that of plasma [1141].
Swallowed nitrite can be reduced to NO in the acid solution of the stomach. Intragastric NO regulates gastric blood flow, mucus production, and host defense. In conditions of very low pH, such as in the stomach, nitrous acid (HNO2) spontaneously generates nitric oxide [1154].
In some organs, nitrate can also be reduced into nitrite. In the liver, xanthine oxidoreductase serves as nitrate reductase. In erythrocytes and vascular endothelial cells, in normal conditions, the activity of xanthine oxidoreductase is minimal, but rises during acidosis and hypoxia [1141].
Nitrate released from skin stores has a substantial concentration in sweat. It is then reduced to nitrite by skin commensals and, then, nitric oxide.
10.3.3 Nitric Oxide and Hypoxic Vasodilation
When an overall regulation is required, both central and local mechanisms provide a coordinated adjustment of blood flow to metabolic demand. When an increased demand is confined to a restricted region, i.e., when a change in metabolic activity concerns a limited number of adjacent groups of working cells, a local vasodilation of the microvascular bed (arterioles), the so-called hypoxic vasodilation (Table 10.5),38 occurs in response to decreased oxygen saturation of hemoglobin to promptly raise the local blood perfusion, hence supplying more oxygen, without changes in the upstream arterial condition, such as vascular resistance at a distance from the site of interest, as well as without modifications in the control by the central nervous system and activity of circulating hormones.
Table 10.5
Types of mechanotransduction in different compartments of the arterial bed. Autoregulation associated with the myogenic tone results from paradoxical vasoconstriction (smooth mucle cell contraction) when the local arterial pressure heightens and vasodilation when the flow rate rises.
Compartment | Effect |
---|---|
Large arteries | Vasodilation in response to prolonged increase |
of intraluminal pressure | |
(direct and endothelium-mediated response of VSMCs) | |
Resistive arteries | Autoregulation (maintenance of constant flow rate) |
Calcium sparklets and membrane potential change | |
in both endothelial and smooth muscle cells | |
Arterioles | Hypoxia-triggered vasodilation |
on increased local metabolism | |
(Snitrosothiol-based process) |
Hypoxic vasodilation relies on a pathway, in which the signal is the decreased ATP production that results from a local lack in oxygen. The sensor localizes to hemoglobin in erythrocytes, as the response is correlated to Hb–O2 saturation, but not tissue partial pressure in oxygen.
In addition to hemoglobin, other factors, such as ATP, adenosine, vasoactive peptides, CO2, as well as H + and K + cations can contribute to the hypoxic vasodilation [1158].
The distribution of blood flow in arteriolar networks according to metabolism of active cells and regulation of capillary perfusion by arterioles is also controlled by sympathetic nerve activity [1161]. Sympathetic constriction of proximal arterioles and feed arteries can restrict functional hyperemia on resting territories, whereas dilation prevails in distal arterioles that irrigate working tissues to promote oxygen extraction. In addition, venules yield feedback from metabolic state of drained tissues to nearby arterioles via the production of vasodilators.
10.3.3.1 Role of Erythrocyte and Hemoglobin
Hemoglobin tetramer, during its transformation from the relaxed state (R state) to tense state (T state), releases NO bound to a cysteine in its β chain (Cysβ93). The latter is subsequently carried as low-molecular-weight Snitrosothiols, such as Snitrosocysteine (CysNO) and Snitrosoglutathione (GSNO). These agents have longer life duration than free NO and are protected from scavenging by hemoglobin hemes.
Owing to erythrocytic Hb, erythrocytes consume and produce NO using nitrite. Nitric oxide reacts with oxyHb slightly faster than with deoxyhemoglobin. At relatively low oxygen partial pressures, NO consumption is thus slightly less efficient, but NO production rises due to the reaction of nitrite and deoxyhemoglobin. Therefore, relatively more NO is produced than consumed as erythrocytes deoxygenate [1154].
Erythrocytes actually release Snitrosothiols into the blood stream during hypoxia [1162].39 SNitrosohemoglobin (HbSNO) binds to solute carrier superclass anion exchanger SLC4a140 that facilitates electroneutral anion exchange. By transnitrosylation, the NO group then transfers to Cys in the cytoplasmic N-terminus of SLC4a1. On the other hand, ATP-binding cassette protein can transport Snitrosoglutathione.
SNitrosohemoglobin in erythrocytes can elicit a relaxation of vascular smooth muscle cells via cGMP andCa
-dependent K + channels andCa
ATPase, independently of endothelium-derived NO and nitrite [1162]. In addition, oxyhemoglobin (HbO2) can be oxidized by NO to yield methemoglobin (metHb, i.e., Fe 3 + Hb that cannot carry oxygen, unlike normal
Hb, and NO3 − [1163]. Afterward, NADH-dependent methemoglobin reductase converts methemoglobin into hemoglobin. In systemic vessels,41 Snitrosohemoglobin intervenes in hypoxic vasodilation with a time scale of second.42



10.3.4 Reduction–Oxidation Reaction Products of Nitric Oxide
Reduction (gain of electron) and oxidation (loss of electron) of nitric oxide give redox forms: (1) hyponitrite anion (NO − ), or nitroxyl anion and (2) nitrosonium cation (NO + ), or nitrosyl cation, respectively (Table 10.6). The uncharged free radical form of nitric oxide (NO ∙ ) that activates Ca
-activated K + channels (K Ca ) repolarizes smooth muscle cells at a lower extent than nitroxyl [1165].

Table 10.6
Reduction and oxidation of nitric oxide into hyponitrite (or nitroxyl) anion (NO − ) and nitrosonium (or nitrosyl) cation (NO + ), respectively.
Reduction | NO + e − | → | NO − |
---|---|---|---|
Oxidation | NO | → | NO + + e − |
Nitric oxide yields several different redox forms. Its 1-electron reduction product, protonated nitroxyl (HNO) and nitroxyl anion (NO − ),43 causes a hyperpolarization of vascular smooth muscle cells and repolarization as well as vasorelaxation of contracted cells in resistance arteries, as it acts onvoltage-dependent K + channels (KV) via the sGC–cGMP pathway [1165].
The endogenous production of HNO may occur via various pathways. Nitroxyl can be synthesized by NOS, particularly in the absence of the cofactor tetrahydrobiopterin or after oxidation of the NOS intermediates N ω hydroxy Larginine and hydroxylamine [1166].44 Moreover, HNO can be produced from the reduction of NO ∙ by mitochondrial cytochrome-C, xanthine oxidase, and hemoglobin.
Nitroxyl anion induces vasodilation in association with the formation of iron–nitrosyl complexes and the conversion of NO − to NO ∙ [1167]. Protonated nitroxyl is an endothelium-derived relaxing and hyperpolarizing factor in resistance mesenteric arteries of rats and mice, but with different potency order in these 2 rodent species with respect to other vasodilatory agents (NO ∙ ∼ HNO > EDHF in mice and EDHF > HNO ∼ NO ∙ in rats; EDHF: endothelium-derived hyperpolarizing factor) [1166].
Superoxide dismutase (SOD) rapidly scavenges superoxide (O2 − ), thereby prolonging the vasorelaxant effects of nitric oxide. Enzyme SOD supports a reversible reduction of NO to NO − [1163] (Table 10.7). Although peroxynitrite (ONOO − ) formed by dismutation of NO with O2 − can decompose into NO2 and OH ∙ in the absence of SOD or may convert to NO2 + in its presence, nitrate (NO3 − ) results from peroxynitrite.
Table 10.7
Metabolites of nitric oxide (Source: [1163]). Reaction of NO with O2 − (reactions 1a and 1b) and O2 (reactions 2 and 3). Reaction 1 is irrelevant in situations where a high local concentration of O2 − occurs, such as in the immediate vicinity of activated macrophages and neutrophils. Reaction 4 is analogous to the normal reaction of SOD, in which
SOD accepts an electron from O2 − to produce O2 and converts into Cu + SOD. A proton donation from SOD may facilitate a reduction of NO to HNO (reaction 5). The reduction (reverse reaction from NO to NO − in reaction 4) is analogous to another normal reaction of SOD with O2 − that yields H2O2 (reaction 6).


In addition, Snitrosothiols (RSNO; R denotes an organic group; R–N = O: nitroso functional group),45 or thionitrites, that serve as donors of the nitrosonium ion NO + can react with other thiol species to yield HNO at physiological pH [1166]. Activity of NO actually results from NO and Snitrosothiol derivatives.
Under hypoxia, nitrite ions (NO2 − ) may release nitric oxide that causes potent vasodilation. Nitrite may also react with deoxyhemoglobin in venous blood to generate nitrosylated iron (
NO) [1155]. The subsequent conversion to SNO results from oxygenation at neutral pH in the lung vasculature and acidification in the gut. Endogenous Snitrosothiols are much more potent vasodilators than nitrites.

In platelets, both HNO and NO ∙ exert an anti-aggregating effect.
In cardiomyocytes, HNO enhances Ca
cycling and sensitivity of Ca
handling proteins. Nitroxyl anion has positive inotropic and lusitropic effects. Cardiac inotropic effect of nitroxyl anion is mediated by the neuromodulator calcitonin gene-related peptide via the release of CGRP from non-adrenergic, non-cholinergic fibers (NANC) [1167]. Improved left ventricular contractility is associated with a concomitant selective venodilation (without any change in arterial resistance). Therefore, HNO is called an inodilator.46


10.3.5 NO Transfer
Nitric oxide is a small hydrophobic molecule that can cross cell membranes without necessarily using carriers, unlike the gaseous molecules oxygen and carbon dioxide that use gas transporter (Vol. 3 – Chap. 4. Membrane Compound Carriers). Nevertheless, aquaporin Aqp1 carries hydrophobic NO and O2 molecules.
Nitric oxide can diffuse from its synthesis site to surrounding cells, where it activates soluble guanylate cyclase (sGC; Vol. 3 – Chap. 6. Receptors). Erythrocytes yield a major sink for NO in the flowing blood that creates a diffusion gradient between irrigated tissues and blood.
The diffusion coefficient of NO in water at 37 ∘ C is slightly faster than that of oxygen and carbon dioxide [1168]. This short-lived molecule (half-life of ∼ 1 s) diffuse rapidly with an average molecular velocity of
[100 m/s], following a trajectory in solution determined by
[109] collisions per time unit (1 s). Due to its short life,47 Agent NO travels a relatively short averaged distance. Its action is thus limited to cells close to the source of production.


Nitric oxide mainly signals via soluble guanylate cyclase that produce cGMP, a second messenger.48 Like hemoglobin, soluble guanylate cyclase contains heme protoporphyrin-9 with iron in the ferrous form that binds NO with high affinity.49 In turn, cGMP activates cGMP-dependent protein kinase-G. The greater the local NO concentration, the larger the amount of cGMP synthesized.
10.3.6 NO Effects
Nitric oxide transmits information in different modes. The first triggered pathway relies on soluble guanylate cyclase (sGC)50 that synthesizes cyclic guanosine monophosphate. Soluble guanylate cyclases that pertain to the heme nitric oxide- and oxygen-binding (HNOX) proteins are heterodimeric hemeprotein.51 Two isoforms exist (α1β1 and α2β1) that have similar enzymatic properties. At least in some tissues, these isoforms can compensate for each other. Endogenous modulators of sGC include ATP, GTP, Ca
, Mg
, and protein kinases and phosphatases, among other interactors: [1169].52 Nitric oxide exerts its effects only partly by binding to guanylate cyclase receptors, thereby priming cGMP accumulation in target cells (Vol. 3 – Chap. 6. Receptors).53


Second messenger cGMP can modify the activity of ion channels (e.g., cyclic nucleotide-gated channels), protein kinase-G, protein phosphatases, and phosphodiesterases,54 especially cGMP-specific PDE5 isoform. Substrates of protein kinase-G include inositol trisphosphate receptor-associated PKG1 substrate (IRAG),55BK channels, PDE5, cerebellar G substrate,56 vesicle-associated membrane protein (VASP), and telokin [10]. The NO–GCase–cGMP pathways (Table 10.8) are involved in smooth muscle relaxation, synaptic plasticity, and cardiac hypertrophy. Nitric oxide thus participates in the regulation of the vasomotor tone (Vol. 5 – Chaps. 8. Smooth Muscle Cells and 9. Endothelium), as well as ion conductance, glycolysis, immunity, neurotransmission, and cell apoptosis.
Table 10.8
Signaling by the NO–sGC–cGMP pathway (Source: [1169]; CNG: cyclic nucleotide-gated channel; IRAG: IP3R-associated PKG1β substrate; MLCP: myosin light-chain phosphatase; PDE: phosphodiesterase; pGC: particulate guanylate cyclase; PKG: cGMP-dependent protein kinase-G (or cGK); sGC: soluble guanylate cyclase; VASP: vasodilator-stimulated phosphoprotein). Three types of cGMP-binding proteins transduce the cGMP signal: (1) cGMP-modulated cation channels; (2) cGMP-dependent protein kinases; and (3) cGMP-regulated phosphodiesterases that degrade cGMP and/or cAMP messengers. Membrane-bound PKG2 mediates effects of pGC-derived cGMP on intestinal electrolyte transport and bone formation. Cytosolic PKG1, which has 2 isoforms (PKG1α–PKG1β) transduces many effects of sGC-derived cGMP messenger. Enzyme PKG1 stimulates sumoylation of the ETS-like transcription factor ELk1, thereby derepressing smooth muscle-specific gene promoters.
Target | Pathway |
---|---|
Effect | |
Ion channel | NO–sGC–cGMP–CNG–Ca ![]() |
Phosphodiesterases | NO–sGC–cGMP–PDE–cAMP/cGMP |
Cell growth and differentiation | |
Inhibition of platelet aggregation | |
Protein kinase-G | NO–sGC–cGMP–PKG1α |
Growth of vascular smooth muscle cell | |
NO–sGC–cGMP–PKG1α–MLCP | |
Smooth muscle relaxation | |
NO–sGC–cGMP–PKG1β–IRAG–Ca ![]() | |
Inhibition of intracellular Ca ![]() | |
Smooth muscle relaxation | |
NO–sGC–cGMP–PKG1α/β–VASP | |
Inhibition of platelet adhesion to endothelium | |
NO–sGC–cGMP–PKG1–ELk1 | |
Smooth muscle gene transcription |
Maximal relaxation of airway smooth muscle by nitric oxide that stimulates soluble guanylate cyclase is greater than that produced in response to atrial natriuretic peptide that activates the particulate guanylate cyclase (pGC). Stimulation of pGC causes a bronchodilation exclusively by decreasing the intracellular Ca
concentration, whereas stimulation of sGC decreases both [Ca
] i and Ca
sensitivity, i.e., the force developed for a given Ca
level [1170].




In rat renal glomeruli, inhibitors of inducible nitric oxide synthase NOS2 may also repress the activity of soluble guanylate cyclase, but increase the activity of particulate guanylate cyclase to compensate that of sGC [1171].
Nitric oxide also contributes to the increase in muscle blood flow during exercise after acute administration of ascorbic acid [1172].
Biological effects of NO can also be mediated by modifications of proteins. Nitric oxide can indeed operate via reactive oxygen and nitrogen species, thereby modifying the activity of target proteins via nitrosylation. Nitric oxide actually interacts with various acceptors, such as superoxide radical (O2 − ∙ ), cysteine, glutathione, and transition metal ions:

The S-nitrosylation reaction relies on the transfer of NO from one of the reactive nitrogen species to a thiol group of target proteins (R–S) [10]:

Nitric oxide may reversibly inhibit enzymes with transition metals or with free radical intermediates in their catalytic cycle. In micromolar concentrations, NO reversibly impedes the activity of catalase and cytochrome-P450 [1168]. It can also hinder the action of ribonucleotide reductase used in DNA synthesis that contains a tyrosine radical.
Cells are very sensitive NO detectors, as they are able to respond to tiny concentration of NO (1 pmol) by rising their cGMP content ( ≥ 30 nmol) [1173]. Moreover, they can sense brief NO puffs (100 ms) that yield a peak intracellular NO concentration of about 2O pmol. Therefore, NO is able to signal at extremely low concentrations from a tiny proportion ( ∼ 1%) of cognate activated receptors.
Concentration of nitric oxide influences its effects. At 100 nmol or less, NO activates the cGMP synthesis as well as the protein kinase-G57 and extracellular signal-regulated protein kinase pathways [1174]. At higher concentrations (300–800 nmol), it stimulates the protein kinase-B pathway and hypoxia inducible factor-1α, and stabilizes P53 transcription factor.
Nitric oxide and its messenger cGMP control Ca
homeostasis in a cell-specific manner. In pancreatic acinar cells, nitric oxide potentiates Ca
entry. In hepatocytes, NO causes oscillatory increase in intracellular Ca
level.



In platelets and vascular smooth muscle cells, NO precludes Ca
entry and intracellular store refilling. In cardiomyocytes, NO impedes the activity ofCaV1.2a channel.

Most of NO effects result from its regulation of gene expression.58 The NO pathway supports the activation of the telomerase reverse transcriptase (TERT; i.e., catalytic subunit) during angiogenesis [1174]. In addition, S-nitrosylation of cysteines represses HDAC2 histone deacetylase complex.
The ubiquitin-dependent N-end rule pathway recognizes degradation signals based on a destabilizing N-terminal residue. Oxidation of N-terminal cysteine (before its arginylation) of regulatory proteins requires nitric oxide. In the heart, NO regulates particularly proteolysis of regulator of G-protein signaling RGS4, RGS5, and RGS16 [1175].
Perturbation of the PI3K–PKB–NOS3 cascade pertains to the set of mechanisms responsible for the reduction and dysfunction of bone marrow-derived endothelial progenitor cells in type-2 diabetes mellitus [1176].59
10.3.6.1 NO Activity in Blood and Lymph Vessels
Once bound to the heme iron of soluble guanylate cyclase, nitric oxide ultimately causes smooth muscle relaxation [1177, 1178] (Table 10.9). Subtype NOS3 is phosphorylated (activated) by Src kinase, protein kinases PKA, PKB, and PKC, and AMP-activated protein kinase. In endothelial cells, NOS3 is also activated via angiotensin-2 AT1 and bradykinin B2 receptors. Phosphorylation of NOS3 by the Src–PI3K–PKB axis causes AT1–NOS3 dissociation and subsequent increase in NO synthesis [1185].
Target | Function |
---|---|
Guanylate cyclase | cGMP formation |
NADPH oxidase | Enzyme inhibition |
Tissue plasminogen activator | Vasodilation, platelet inhibition |
Ca ![]() | Vasodilation |
Cyclooxygenase-2 | Prostaglandin synthesis |
Cytoskeleton proteins | Dysfunction |
The NOS–NO signaling axis is vasoprotective, preventing the occurrence of pulmonary arterial hypertension. In addition, nitrite anion, a complementary source of NO, yields another adaptive, vasoregulatory pathway in the pulmonary and systemic vasculatures. Multiple nitrite reductases convert nitrite to NO, such as deoxyhemoglobin and myoglobin in the circulation and heart, respectively, and xanthine oxidoreductase in the lung parenchyma [1159].
In the heart, abundant myoglobin act as a nitrite reductase.60 At least in the murine vasculature, myoglobin (at very low concentrations in vascular smooth muscle cells) contributes significantly to nitrite-induced vasodilation [1180].
Angiogenesis after injury and ischemia allows tissue repair. It relies, at least partly, on pro-angiogenic nitric oxide synthesized by NOS3 synthase activated by PKB kinase. Nitric oxide represses anti-angiogenic thrombospondin-2 [1181]. Thrombospondins enhance clearance of matrix metallopeptidases MMP2 and MMP9 and interact with plasmalemmal receptors, such as αVβ3-integrin, very low density lipoprotein receptor (VLDLR), thrombospondin receptor CD36 (or scavenger receptor ScaRb3), and integrin-associated protein CD47, another thrombospondin receptor, to inhibit angiogenesis. Conversely, thrombospondin-1 impedes the ability of NO to activate soluble guanylate cyclase in vascular smooth muscle cells and NOS3 activity by blocking phosphorylation (Ser1176), hence endothelial-dependent arterial relaxation [1182].
NO in Lymphatics
Immune surveillance relies on antigen-presenting cell transport in lymphatics to bring antigens in draining lymph nodes. Initial lymphatics are endothelium-lined conduits without perivascular cells (pericytes and smooth muscle cells). They are endowed of overlapping cell junctions forming primary valves in addition to traditional secondary lymph valves [1183]. Fluid transport inside lymphatics relies on surrounding tissue motions that generate lymphatic expansion and compression. On the other hand, collecting contractile lymphatics are equipped with smooth muscle cells and bileaflet valves to carry fluid from initial lymphatics to lymph nodes. Lymphatic transport thus depends on smooth muscle cell contraction, hence on regulators of cell contraction and relaxation. In particular, it is influenced by nitric oxide. Under physiological conditions, NO produced by NOS3 in lymphatic endothelial cells is required for autonomous, periodic contractions of lymphatics [1184]. During inflammation, NO synthesized by NOS2 in bone marrow-derived CD11b + myeloid cells that infiltrate surrounding tissue of contractile lymphatics attenuates lymphatic contraction [1184].
Nitric Oxide, Hemoglobin, and Oxygen Content
Nitric oxide targets heme-containing molecules to form NO–hemoprotein complexes (NO–hemoglobin, NO–myoglobin, NO–sGC, etc.). Nitric oxide binds to hemoglobin about 106 times more tightly than oxygen. Extracellular, but not intracellular, hemoglobin interferes with NO-dependent vasoactivity. A cycle of reversible NO binding to hemoglobin that sequesters NO in erythrocytes and releases it from these cells participates in respiratory gas transport in the tissues. Hypoxia drives more or less simultaneous unloading of NO and oxygen. The relative number of hemoglobin molecules in erythrocytes that carry out the vasoactive function is very small ( ∼ 0.1% of the total amount).
Oxyhemoglobin lowers cGMP levels, thereby impeding effects of endothelium-dependent relaxants acetylcholine61 and bradykinin on isolated rings of bovine intrapulmonary artery and vein, as well as of nitric oxide radical on endothelium-denuded rings.
Nitric Oxide and Natriuretic Peptides
Nitric oxide and atrial natriuretic peptide provoke cGMP synthesis and influence cell functioning via cGMP-dependent protein kinases (PKG) and cGMP-specific phosphodiesterases (for signaling termination), as well as cyclic nucleotide-activated ion channels. They prime 2 different pathways in vascular smooth muscle cells and cardiomyocytes owing to spatial segregation of cGMP signaling [1186].
Natriuretic peptides ANP, BNP, and CNP target their specific plasmalemmal receptor guanylate cyclases NPR1 (or pGCa) and NPR2 (or pGCb) to cause sustained, compartmentalized cGMP synthesis.62 Nitric oxide activates cytosolic soluble guanylate cyclases sGCα1 and sGCβ1 to transiently produce cGMP messenger.
In aortic vascular smooth muscle cells, nitric oxide lowers the expression of Gi-coupled natriuretic peptide receptor NPR3 via a cGMP-independent, MAPK-dependent pathway [1187]. Decreased expression of Gαi subunit of G protein is delayed with respect to that of NPR3 receptor. Phosphorylated extracellular signal-regulated kinases ERK1 and ERK2 increases and then decreases.
Vascular Cell Fate
Nitric oxide inhibits growth and migration of vascular smooth muscle cells. On the other hand, NO stimulates endothelial cell proliferation and prevents endothelial cell apoptosis. Moreover, nitric oxide provokes apoptosis of vascular smooth muscle cells via reactive nitrogen species. Factor P53 protects vascular smooth muscle cells from NO-induced apoptosis, as it promotes synthesis of anti-oxidant proteins such as peroxiredoxin-3 (PRx3) [1188]. Anti-oxidant PRx3 operates with its specific electron acceptor thioredoxin-2. Transcription factor P53 can also exert its protection via mitogen-activated protein kinase modules and heme oxygenase-2.
Matrix Metallopeptidases
Remodeling of the extracellular matrix requires matrix metallopeptidases. Nitric oxide regulates MMP1, MMP9, and MMP13 [1189]. It controls MMP9 secretion from macrophages via protein modification mediated by reactive nitrogen species, soluble guanylate-cyclase-dependent modulation of the MMP9–TIMP1 balance, as well as MMP1- and MMP13-dependent cleavage of MMP9 enzyme.
Sirtuin
During caloric restriction, sirtuin-1 deacetylase63 decreases arterial blood pressure and provokes vasodilation. Sirtuin-1 deacetylates NOS3 [1190]. Among other possible factors, it reduces both systolic and diastolic blood pressure during long-term reduced caloric intake not only in overweight individuals, but also in healthy subjects.
Tumor vasculature has abnormal structure, organization, and function that compromise both tumor oxygenation and delivery of antitumor agents. Nitric oxide mediates the effects of many angiogenic factors, such as vascular endothelial growth factor, angiopoietin-1, and sphingosine 1-phosphate. It can also induce the expression of angiogenic factors, such as VEGF and FGF2. Elimination of nitric oxide production from tumor cells by inhibiting NOS1 creates a perivascular NO gradient that normalizes the tumor vasculature [1191].
10.3.6.2 NO Activity in the Heart
In the heart, NO is an important regulator of coronary perfusion and cardiac contractility [1192]. Both NOS1 and NOS3 are constitutively expressed in cardiomyocytes. In addition, NOS3 abounds in endothelial and endocardial cells and NOS1 in both adrenergic and cholinergic nervous fibers.64 In fact, NO is synthetized by all cardiac cell types.
In cardiomyocytes, NOS lodges in distinct subcellular compartments.65 Synthase NOS3 resides in sarcolemmal and T-tubular caveolae, associated with myocyte-specific caveolin-3.66 It is also observed in the sarcoplasmic reticulum, associated with ryanodine receptors, and mitochondria. Enzyme NOS1 localizes to the sarcolemma and sarcoplasmic reticulum;67 NOS1α in mitochondria.
Nitric oxide synthesized in cardiomyocyte exerts intracrine effects as well as paracrine action on adjacent cardiomyocytes. Nitric oxide generated from non-cardiomyocyte sources (endocardial cells, coronary vascular cells, autonomic neurons, fibroblasts, and blood cells) operates on cardiomyocytes.
In the heart, nitric oxide produced by endothelial cells favors the perfusion via vasodilation and prevention of platelet aggregation. Nitric oxide in endothelial cells subjected to mechanical and chemical stimulators increases the intracellular calcium level that activates NOS3 enzyme. It indeed promotes the binding of Ca
–calmodulin to NOS3 and activation of PI3K and, subsequently, PKB kinase. Nitric oxide produced by cardiomyocytes regulates the force and rate of contraction. Moreover, noradrenaline and acetylcholine released from autonomic nerve endings stimulate their respective receptors to activate NOS3 in cardiomyocytes (Fig. 10.1).

Effects of NO on Cardiac Ion Carriers
Nitric oxide modulates the activity of cardiac ion channels involved in the genesis of the cardiac action potential and can exert anti-arrhythmic effects via cGMP-dependent (protein kinase-G and phosphodiesterases) and cGMP-independent mechanisms (S-nitrosylation and direct effects on G proteins) [1193].
In the sarcolemma, NOS1 interacts with Na + –K + ATPase and plasma membrane Ca
–calmodulin-dependent Ca
ATPase PMCA4b. In the sarcoplasmic reticulum, NOS1 is associated with ryanodine receptor RyR2 and Ca
ATPase SERCA2 to regulate intracellular calcium cycling and excitation–contraction coupling.



Sodium Carriers
In atrio- and ventriculomyocytes, NO either inhibits inward Na + current without altering channel conductance and kinetics via both PKG and PKA, or causes a late Na + flux via S-nitrosylation of cardiacNaV1.5 channel encoded by the SCN5A gene [1193]. In the sarcolemma, NaV1.5 complexes with NOS1 and its inhibitor PMCA4b, as well as α1-syntrophin, a scaffold for NOS1, PMCA4b, and α1-syntrophin.
On the other hand, NO does not seem to influence sarcolemmalNa + –Ca
exchanger. Both NOS1 and NOS3 colocalize with caveolin-3 and Na + –K + ATPase in the sarcolemma of cardiomyocytes. Nitric oxide increases the activity of these pumps.

Nitric oxide exerts a cGMP-dependent stimulation on pacemaker, hyperpolarization-activated, cyclic nucleotide-gated, HCN1 to HCN4 channels that convey an inward, mixed Na + and K + flux.68
Calcium Channels
Nitric oxide can increase, decrease, or produce a biphasic effect onCaV1.2 channel, according to its concentration and the context [1193]. S-nitrosylation reduces its functioning. Nitric oxide regulates β-adrenoceptors that activates CaV1.2 via the ACase–cAMP–PKA pathway. Adaptor NOS1AP inhibits CaV1.2 channel.
Cardiac excitation–contraction coupling relies on ryanodine receptors. S-nitrosylation supports channel activation.69 In addition, NOS3-derived NO enhances the open probability of RyRs and frequency of Ca
sparks in response to myocardium stretch via the PI3K–PKB–NOS3 pathway [1193]. In rat ventriculomyocytes, NO influences RyR function according to the level of β-adrenergic stimulation and the state of PKA activation [1193].70

Potassium Channels
In human atrial and ventricular myocytes, KV4.3 channel is responsible for early rapid repolarization (phase 1 of the action potential), thereby influencing the activation of other ion carriers that control repolarization (CaV1.2 and delayed rectifier K + channels). Nitric oxide inhibits KV4.2 and KV4.3 channels via PP2 phosphatase and PKA kinase [1193].
Ultrarapid delayed rectifier KV1.5 in human atriomyocytes is repressed by NO via activation of the GC–cGMP–PKG pathway and S-nitrosylation [1193]. Rapid delayed rectifier KV11.1 that contributes largely to phases 2 and 3 of the repolarization in most nodal cells and cardiomyocytes can be inhibited, in particular via radical oxygen species. Slow delayed rectifier KV7.1 that activates slowly during depolarization and deactivates slowly during repolarization and are involved in the late repolarization phase also can be S-nitrosylated.
Inward rectifiers KIR2.1 to KIR2.3 works over a limited range of membrane potentials ( − 30 to − 40 mV), thereby acting in the late phase 3. In human atriomyocytes, NO augments the activity of these channels, thereby shortening the final phase of repolarization.71
Muscarinic M2 receptor stimulates acetylcholine-activated KIR3.1 to KIR3.4 channels in the sinoatrial and atrioventricular nodes and atriomyocytes. Nitric oxide may potentiate the increase in
current primed by acetylcholine [1193].

ATP-sensitive KIR6.1 and KIR6.2 in the sarcolemma and mitochondria couple cell metabolism to membrane potential and exert a cardioprotection during ischemia. In ventriculomyocytes, NO enhances the
current via the GCase–cGMP–PKG pathway.


Fig. 10.1
Enzymes NOS1 and NOS3 cooperate to regulate the sympathovagal balance in the heart (Sources: [1179, 1192, 1198, 1199]). Isoform NOS1 acts in the parasympathetic and sympathetic endings. It potentiates acetylcholine release and hampers noradrenaline. release in the synaptic cleft. Isoform NOS3 (eNOS) is activated in cardiomyocytes by both stimulated muscarinic cholinergic and β3-adrenergic receptors. Activated NOS3 in the cardiomyocyte opposes adrenergic activity, but reinforces vagal input. Catecholamine stimulation of β3-adrenoceptors of cardiomyocytes activates NOS3 via Gαi, which increases cGMP level and leads to negative inotropy. Colocalized NOS3 and ryanodine channels RyR2 (RC2) in the T-tubule–sarcoplasmic reticulum junction favor calcium influx. Messenger cGMP opposes cAMP-associated positive inotropy because it activates phosphodiesterase PDE2 for cAMP degradation, although cGMP can potentiate cAMP effects via inhibition of PDE3 enzyme. Activation of PKG reduces the CaV1.2 activity. Protein kinase-G also decreases myofilament sensitivity to calcium, thereby promoting relaxation. Enzyme NOS1 hinders CaV1.2 channels (VGCC), but promotes sarcoplasmic reticulum calcium ATPase (SERCA). Interstitial NO acts on soluble guanylate cyclase (sGC) for cGMP production, and activates PKG1 kinase. Activated PKG1 inhibits CaV1.2a channels. Enzyme NOS2 triggered by cytokines and other inflammatory mediators in the cardiomyocyte activates the cGMP–PKG pathway, and hence NFκB, leading to TNFα synthesis. The latter upregulates NOS2 in cardiomyocytes.
In guinea pig ventriculomyocytes,2-pore domain K + channels TALK1 (K2P16.1) and TALK2 (K2P17.1) generate an outward current during ischemic preconditioning. Nitric oxide may assist in the protection against prolonged ischemia [1193].
Effects of NO on Heart Intrinsic Properties
In the cardiomyocyte, the regulation of intracrine nitric oxide signaling depends on the location (sarcolemma or sarcoplasmic reticulum) of NO synthase isoforms (NOS1 and NOS3) [1194]. Synthases NOS1 and NOS3 have opposite effects on Ca
influx. Nitric oxide inhibits β-adrenergic-induced inotropy after compartmentation of NOS3 in caveolae, hamperingCaV1 channels.72 On the other hand, NOS1 associates with ryanodine receptors of the sarcoplasmic reticulum and then stimulates Ca
release.


Isoform NOS3 facilitates the electromechanical coupling in response to sarcomere stretch. It actually sustains length-dependent slow increase in calcium transient and force generation in stretched fibers (Anrep effect). Isozyme NOS1 hinders calcium current through CaV1 and enhances calcium reuptake into the sarcoplasmic reticulum by SERCA pumps (Fig. 10.1). Hence, NOS1 and possibly paracrine NO from adjoining endothelial cells promote cardiomyocyte relaxation and thereby ventricular filling that increases stretch. Moreover, NOS1 and NOS3 attenuate β1- and β2-adrenergic positive inotropy and chronotropy. The NOS3 enzyme also potentiates acetylcholine activity. The NOS synthases reinforce pre- and postsynaptic vagal control of the cardiac contraction. They thus protect the heart against excessive stimulation by catecholamines. In an ischemic myocardium, NOS1 augments the effect of constituve NOS. Last but not least, NOS modulates oxygen consumption, promotes free fatty acids rather than glucose oxidation, struggles against oxidative stresses, prevents apoptosis at low concentrations, and acts in adaptive and regenerative processes.
Cyclic adenosine (cAMP) and guanosine (cGMP) monophosphate have opposing effects in cardiomyocytes.73 A high NO concentration increases the cGMP level leading to negative inotropy by a PKG-dependent reduction in myofilament responsiveness to calcium. (Calcium ions remain available.) Low NO concentration increases cAMP level by adenylate cyclase activation, leading to positive inotropy [1195]. The negative cGMP effects in cardiomyocytes are mediated by cGMP-gated ion channels, protein kinase-G, and phosphodiesterases. The cGMP-stimulated cAMP phosphodiesterase PDE2 and cGMP-inhibited cAMP phosphodiesterase PDE3 are regulated by the intracellular cGMP concentration. Enzyme PDE3 is inhibited by an increase in cGMP level that raises the cAMP level. Protein kinase-A then activates calcium channels, countering PKG effects. On the other hand, cGMP can stimulate PDE2, thereby reducing the cAMP level and PKA activity. Interaction between cGMP and cAMP signaling pathways is impaired in failing cardiomyocytes [1196].
Protein kinase-A phosphorylates CaV1 channels to increase calcium influx using calcium-induced calcium release, thereby enhancing cardiomyocyte contraction [1179]. Messenger cGMP stimulates PKG that inhibits CaV1 channels and activatesBK channel and myosin light-chain phosphatase.
Adaptor NOS1AP 74 is a NOS1 regulator that interacts with NOS1, but not NOS3 subtype. It accelerates cardiac repolarization (short duration of action potential), as it inhibits CaV1.2 channels and enhances the activity of rapid delayed rectifier potassium channels (KV11.1; i Kr current) by cGMP-dependent or -independent pathways in guinea pig ventriculomyocytes [1197]. Adaptor NOS1AP directs NOS1 to specific target proteins.
Nitric oxide acts on soluble guanylate cyclase and triggers the cGMP–PKG pathway. Protein kinase-G1 then inhibits CaV1.2a channels [1198]. Muscarinic receptor stimulation does not act on this cascade but stimulates calmodulin-dependent cardiac nitric oxide synthase. Muscarinic inhibition occurs rather via inhibition of cAMP signaling via Gαi2-dependent inhibition of adenylate cyclase. Stimulation of β1- and β2-adrenergic receptor produces positive inotropy via Gαs-mediated activation of adenylate cyclase that augments cAMP levels (Fig. 10.1).
Nitric oxide produced by inducible NOS2 in all cardiac cell types, including interstitial macrophages, under exposure to cytokines and other inflammatory mediators, exerts para- and autocrine effects. Large NO concentrations decrease the contraction of cardiomyocytes and vascular smooth muscle cells. Nitric oxide produced by NOS2 also causes apoptosis of various cell types. In the cardiomyocyte, NO provokes TNFα synthesis using the cGMP–PKG pathway. Agent TNFα induces NOS2 expression and yields a positive feedback inflammatory loop [1199].
Low myocardial NO availability during coronary vascular inflammation, LArg methylation that produces NOS inhibitors, and low concentration of NO metabolites in coronary veins reduces NO lusitropic effect and restrains left ventricle filling [1200].
Nitric oxide operates in differentiation of embryonic stem cells into myocardial cells [1201]. Nitric oxide and its receptor, soluble guanylate cyclase, activate expression of cardiac genes (e.g., myosin light chain MLC2 and Nkx2-5 factor).
Insulin exerts a cardiovascular protection via the PI3K–PKB–NOS3 pathway in vascular endothelial cells and cardiomyocytes [1202]. Insulin then supports NO production by NOS3 enzyme. Nitric oxide causes vasodilation on the one hand and exerts anti-apoptotic and prosurvival effects on the other (Table 10.10).
Table 10.10.
Insulin-triggered pathways for cardioprotection (Source: [1202]; ↑: increase; ⊖ : inhibition). Insulin binds to insulin receptor and activates 2 main signaling cascades: PI3K–PKB–NOS3 and the mitogen-activated protein kinase (MAPK) module. Activated insulin receptor (IR) phosphorylates insulin receptor substrate IRS1, leading to the binding and activation of phosphatidylinositol 3-kinase (PI3K), subsequently of phosphoinositide-dependent protein kinase PDK1 and protein kinase-B (PKB) that phosphorylates NOS3. Production of NO then rises within mn. In addition, PKB inhibits GSK3β and activates target of rapamycin (TOR) and P70 ribosomal S6 kinase. Insulin stimulates K + reuptake through Na + –K + ATPase and glucose uptake via GluT4 transporter for glycolytic energy production. The insulin–MAPK pathway regulates the secretion of the vasoconstrictor endothelin-1 from endothelium.
Pathway | Effect |
---|---|
IR–IRS1–PI3K–PDK1–PKB–NOS3–NO | Vasodilation, coronary perfusion ↑ |
Cell survival | |
⊖ oxidative stress | |
⊖ inflammation | |
IR–IRS1–PI3K–PDK1–PKB–GluT4 | Glucose endocytosis |
IR–IRS1–PI3K–PDK1–PKB–TOR | Protein synthesis |
IR–Ras–MAPK | Cell proliferation |
Endothelin-1-mediated vasoconstriction |
10.3.6.3 Nitric Oxide and Nephron Transport
Nitric oxide influences several functions of the kidney: salt and fluid reabsorption, renal hemodynamics, and renin secretion. Three nitric oxide synthase isoforms (NOS1–NOS3) are expressed by nephron segments, in addition to renal vessels.
In the kidney, nitric oxide has natriuretic and diuretic effects, as NO modulates electrolyte and water transport in different segments of the nephron (Table 10.11). The natriuretic and diuretic effects of NO are not actually accompanied by proportional changes in glomerular filtration rate or renal blood flow. In the proximal tubule, NO inhibitsNa + –H + exchanger that is responsible for HCO3 − reabsorption and basolateral Na + –K + ATPase that yields energy required for Na + -coupled transport [1203]. In the thick ascending limb, NO impedes the activity of Na + –K + –2Cl − cotransporter that reabsorbs NaCl and Na + –H + exchanger that uptakes most of HCO3 − , which escapes proximal reabsorption, whereas it stimulates apical K + channels that recycle K + across the apical membrane necessary for Na + –K + –2Cl − cotransport and positive luminal potential to provide driving force for paracellular transport of Ca
and Mg
ions. In the collecting duct, NO hinders H + ATPase. In addition, NO reduces vasopressin-stimulated water reabsorption.


Table 10.11.
Nitric oxide effect on nephron transport (Source: [1203]). Proximal tubule reabsorbs 50 to 60% of filtered inorganic solutes and water, whereas organic solutes (glucids, amino acids, and other metabolites) are completely reabsorbed. Solutes are transported by apical Na + -coupled cotransporters (e.g., Na + –glucose, Na + –PO
, and Na + –amino acid cotransporters) or exchangers (Na + –H + exchanger). Water is reabsorbed by diffusion due to the osmotic gradient generated by active solute transport. Thick ascending limb reabsorbs 25 to 30% of filtered salt, but is water impermeable. Cortical collecting duct controls Na + absorption by epithelial (apical) Na + channels (ENaC) and basolateral Na + –K + ATPase, as well as K + excretion by luminal and basolateral K + channels. Water transport that is low is stimulated by arginine vasopressin that augments apical aquaporin number. Cortical collecting ducts also secrete either H + or HCO3 − whether α- or β-intercalated cells predominate. NO does not seem to influence outer medullary collecting duct transport. Inner medullary collecting duct is the last site that regulates salt and water excretion in urine. Na + reabsorption in this segment occurs mainly by apical epithelial Na + channels and basolateral Na + –K + ATPase.

Nephron segment | Ion and water flux |
---|---|
Proximal tubule | Decrease in water and HCO3 − flux |
Inhibition of Na + –K + ATPase | |
Inhibition of type-3 Na + –H + exchanger | |
Thick ascending limb | Decrease in Cl − and HCO3 − flux |
Inhibition of type-3 Na + –H + exchanger | |
Inhibition of Na + –K + –2Cl − cotransporter | |
Stimulation of K + channel | |
Cortical collecting duct | Decrease in water and Na + flux |
Inhibition or stimulation of ENaCs | |
Inhibition or stimulation of K + channel | |
Inner medullary collecting duct | Decrease in Na + flux |
Inhibition of H + ATPase |
Nitric oxide action on nephron transport depends not only on direct effect, but also indirect effect via renal blood flow. Moreover, both nephron cells and neighboring endothelial cells produce NO that operates on nephron with a delay that depends on diffusion time necessary to reach NO targets. Nitric oxide synthesis is regulated. Nitric oxide release can be triggered by many substances such as acetylcholine.
10.3.7 Interaction between Nitric Oxide and Hydrogen Sulfide
Nitric oxide cooperates with another gaseous mediator — hydrogen sulfide (H2S) — in various organs, in particular the heart. Nitric oxide donors can enhance H2S production in smooth muscle cells. Hydrogen sulfide exerts its cardioprotective effect, at least partially, by activating NOS1 and NOS3 [1208].
Owing to its strong reducing capability, H2S may reduce NO to form nitroxyl (HNO), a thiol-sensitive molecule.75 The latter has positive inotropic and lusitropic effects [1208].76 Nitroxyl can operate independently of the cAMP–PKA and cGMP–PKG pathways, as well as β-adrenoceptor. Nitroxyl enhances calcium release from the sarcoplasmic reticulum, as it augments the open probability of ryanodine receptors. It also activates SERCA pumps by S-glutathiolation (Cys674) [1208].
10.4 Hydrogen Sulfide
Endogenous gaseous signaling molecules that are produced at micromolar levels include carbon monoxide, nitric oxide,77 and hydrogen sulfide (H2S).78 They have vasculoprotective effects.
10.4.1 Hydrogen Sulfide Production
Hydrogen sulfide is synthesized from homocysteine metabolism by cystathionine β-synthase and cystathionine γ-lyase79 in vascular smooth muscle cells as well as in many tissues, such as the brain, liver, kidney, ileum, uterus, and placenta [1209].80 Both enzymes contain heme. Their activity depends on cofactor pyridoxal 5′-phosphate. Calcium–calmodulin-activated cystathionine γ-lyase is predominantly located in the vascular endothelium.
Therefore, the trans-sulfuration pathway convert the risk factor homocysteine to the protectant hydrogen sulfide. The extracellular trans-sulfuration enzymes — cystathionine β-synthase and cystathionine γ-lyase — are secreted by microvascular endothelial cells and hepatocytes, circulate in blood, and produce hydrogen sulfide to avoid damages caused by free homocysteine [1210].
An additional enzyme — 3-mercaptopyruvate sulfurtransferase — coupled with cysteine (aspartate) aminotransferase also produces H2S in the presence of α-ketoglutarate [1209].81 This enzyme localizes to the brain and vascular endothelium (e.g., thoracic aorta).
When supplied with elemental sulfur or inorganic polysulfides, erythrocytes produce H2S. In addition, garlic-derived organic polysulfides are converted by erythrocytes and vascular cells into hydrogen sulfide gas [1211].82
Like for other gaseous mediators NO and CO, H2S production is improved by muscarinic cholinergic stimulation of the vascular endothelium. Muscarinic receptors activates cystathionine γ-lyase via the binding to calcium–calmodulin [1209]. However, the mechanism of action of H2S differ from that of NO and CO that activate soluble guanylate cyclase and increase the intracellular cGMP concentration.
In addition, feedbacks exist among different gasotransmitters that enable the regulation of the action of a given gas by others gaseous mediators [1209].
10.4.2 Hydrogen Sulfide Effects
Endogenous hydrogen sulfide modulates neuronal transmission, participates in the endothelium-dependent vasorelaxation, stimulates angiogenesis, and regulates insulin release.83 It relaxes vascular smooth muscle cells via a possible activation of ion channels, in particularKATP channels, thereby hyperpolarizing the membrane potential. Hydrogen sulfide acts as a neuromodulator and metabolic regulator that lowers metabolism. At normal concentrations, it transiently inhibits mitochondrial respiration in a dose-dependent manner. However, its main function is to preserve mitochondrial function. Many of its effects are mediated by potassium channels that cause K + outflux.
Moreover, H2S has anti-inflammatory and anti-oxidant effects. This endogenous gasotransmitter inhibits leukocyte–endothelial cell interactions. Anti-oxidant H2S gas counteracts oxidative and proteolytic stresses, as it opposes activation of matrix metallopeptidases by reactive oxygen and nitrogen species, increases concentrations of tissue inhibitors of metallopeptidases, and lowers apoptosis and fibrosis in chronic heart failure [1213]. Cytoprotectant H2S reduces intimal hyperplasia (restenosis) [1214] and favors angiogenesis [1215].
10.4.3 Hydrogen Sulfide in Mitochondria
Endogenous H2S can regulate energy production in cells during cell stress to cope with energy demand when oxygen supply is insufficient. In vascular smooth muscle cells under normal conditions, cystathionine γ-lyase localizes to the cytosol. Under stress conditions, this enzyme translocates from the cytosol to mitochondria via 20-kDa translocase of the outer mitochondrial membrane TOM20 [1216].84 Cysteine concentration in mitochondria is approximately 3 times that in the cytosol. Mitochondria can use sulfide as an energetic substrate at low micromolar concentrations to maintain ATP production under stress conditions. Translocation of cystathionine γ-lyase to mitochondria processes cysteine, produces H2S inside mitochondria, and improves ATP production under hypoxia. Hence, at physiological concentrations,85 H2S preserves structural and functional integrity of mitochondria. In the cardiovascular system, H2 can then mitigate ischemia injury.
10.5 NADPH and Dual Oxidases
Nicotinamide adenine dinucleotide phosphate (NADPH) oxidase is a membrane-bound enzyme complex. These enzymes comprise many subtypes with different cellular locations. The NADPH oxidase family actually includes 7 known members, i.e., 5 types of NADPH oxidases (NOx1–NOx5) and 2 types of dual oxidases (DuOx1–DuOx2). Every cell type of the vessel wall contains the reduced form of nicotinamide adenine dinucleotide phosphate (NAD[P]H) oxidases (NOx).
Dual oxidases are sensitive to Ca
ions and intervene in Ca
signaling.


The NADPH oxidases belong to the small set of superoxide-producing enzymes of the vasculature.86 Therefore, they produce reactive oxygen species (ROS) [1217] (Fig. 10.2). Isoforms NOx2 and NOx4 are responsible for basal ROS production in vascular endothelial and smooth muscle cells.

Fig. 10.2
NAD(P)H oxidase (NOx) in vascular cells (Sources: [1217, 1219]. This enzyme type is upregulated in vascular smooth muscle cells by platelet-derived growth factor (PDGF), transforming growth factor-β (TGF), and tumor-necrosis factor-α (TNF), and, in endothelial cells, by angiotensin-2 (ATn2). Stimulation of AT1 receptor (AT1R) by angiotensin-2 activates phospholipase-C (PLC) via G protein. Phospholipase-C produces inositol trisphosphate (IP3) and diacylglycerol (DAG), thereby releasing calcium from intracellular stores and activating protein kinase-C (PKC), respectively. The latter phosphorylates the catalytic NOx subunit. Calcium ions activate phospholipase-A2 (PLA2), which produces lysophosphatidylcholine and arachidonic acid (AA). Arachidonic acid is metabolized into prostaglandins by constitutive (COx1) and inducible (COx2) cyclooxygenases. Angiotensin-2 also activates Src kinase, which participates to the activation of EGF receptor (EGFR) and activates protein tyrosine kinases. Activated phospholipase-D produces phosphatidic acid (PA), which is converted to DAG, subsequently enhancing PKC activity. Reactive oxygen species (ROS) activate Src associated with EGFR receptor. Long-duration exposure of vascular endothelial cells to ω3 fatty acid docosahexaenoate (DHA) causes its incorporation into the plasma membrane. Docosahexaenoate inhibits nuclear factor-κB (NFκB) and, subsequently, COx2 enzyme. Furthermore, it decreases the production of reactive oxygen species by inhibiting NADPH oxidase (NOx), and reduces PKC association to the plasma membrane. Last but not least, NOx is stimulated by pulsatile hemodynamic stress.
Reactive oxygen species are oxygen-derived small molecules, i.e., oxygen radicals, such as superoxide (O2 ∙ − , hydroxyl ( ∙ OH), peroxyl (RO2 ∙ ), alkoxyl (RO ∙ ), and certain non-radicals, such as hypochlorous acid (HOCl), ozone (O3), singlet oxygen (1O2), and hydrogen peroxide (H2O2) [1218]. Reactive oxygen species act on cell growth and apoptosis, and cause vasodilation, as they stimulate protein kinases and inhibit protein Tyr phosphatases to activate various transcription factors.
In phagocytes, external signals stimulate their specific plasmalemmal receptors and then trigger the PI3K–PI(3,4,5)P3 pathway. The latter stimulates NADPH oxidase via Rac GTPase. NADPH oxidase uses NADPH as an electron donor to carry out the first step of ROS formation, i.e., the reduction of oxygen to superoxide radical (O2 − ):
NADPH + 2 O2 → NADP + + H + + O2 − ∙ .
In addition to NOx activation via Rac, the second messengers diacylglycerol and calcium may act via protein kinase-C. The latter phosphorylates P47PhOx, a cytoplasmic component of NADPH oxidase, to assemble the NADPH complex [10].
10.5.1 Structure
Nicotinamide adenine dinucleotide phosphate oxidases comprise a set of transmembrane proteins with 6 transmembrane domains that have binding sites for FAD and NADPH and 4 heme-binding histidines in the third and fifth transmembrane domains to produce ROS by transferring an electron to molecular oxygen. Members of the NOX family represent an important source of reactive oxygen species that oxidize nucleic acids (e.g., DNA), carbohydrates, proteins, and lipids.
NADPH oxidases are composed of several subunits. Nine regulatory components exist, including 2 NADPH oxidase organizer subunits — NOxO1 and NOxO2 (P47PhOx) — 2 NADPH oxidase activator subunits — NOxA1 and NOxA2 (P67PhOx) — as well as 2 Rac isozymes (Rac1 and Rac2). The other subunits encompass P22PhOx, P40PhOx, and GP91PhOx87 (Tables 10.12 and 10.13).
Table 10.12.
Subunits of NADPH oxidases that connect to NOx1 to NOx5 catalytic components (Sources: [76, 1043]; CyB: cytochrome-B245, or cytochrome-B558; CyBL(H)C: CyB light (heavy) chain; NCF: neutrophil cytosol factor; NOxA1: NOx activator; NOxO1: NOx organizer; SH3PXD: SH3 and PX domain-containing protein; SNx: sorting nexin). Phagocyte NADPH oxidase consists of a dimer of transmembrane subunits (GP91PhOx and P22PhOx) associated with 4 cytosolic subunits (P40PhOx, P47PhOx, P67PhOx, and Rac2).
Subunits | Other aliases | Interactors |
---|---|---|
CyBα | CyBLC, | NOxA2, NOxO2, P40PhOx, NOx1/4 |
P22PhOx | ||
CyBβ | CyBHC, NOx2, | CyBα, NOxA2, Rac1, |
GP91PhOx, GP91-1 | IQGAP1, actin-γ1 | |
NOxA1 | NCF2L, P51NOx, | NOxO1, NOx1, Rac2, |
P67PhOxL | PKCγ, 14-3-3ζ | |
NOxA2 | NCF2, | NOxO2, P40PhOx, Rac1/2, |
P67PhOx | CDC42 | |
NOxO1 | P41NOx, P41NOxA/B/C, | CyBα/β, NOxA1, NoxO1, |
SH3PXD5, SNx28 | TβR1, | |
NOxO2 | NCF1, NCF1a, | |
P47PhOx, SH3PXD1a | ||
P40PhOx | NCF4, SH3PXD4 | CyBα, NOxA2, NOxO2, |
PKCδ, coronin-1A | ||
Rac1 | ||
Rac2 |
Table 10.13.
Composition of NADPH oxidase isoforms (Source: [1218]). Component P22PhOx is essential for NOx activity; it complexes with other subunits to form NOx oxidases (NOx1–NOx3). In general, the organizer subunit of NOx1 and NOx3 is NOxO1, and that of NOx2 is NOxO2 (P47PhOx); the activator subunit for NOx1 is NOxA1 and that for NOX2 is NOxA2 (P67PhOx). Isotype NOx3 may not need an activator subunit. Isozyme NOx4 does not require cytosolic subunits. Isozymes NOx5, DuOx1, and DuOx2 are activated by Ca
ions; they do not seem to need subunits. Production of P22PhOx subunit is controlled by AP1 and NFκB transcription factors.

Catalytic | Associated regulatory |
---|---|
subunit | subunits |
NOx1 | P22PhOx, NOxO1, NOxA1, Rac1 |
NOx2 | P22PhOx, NOxO2, NOxA2, Rac, |
possibly CyBβ | |
NOx3 | P22PhOx, NOxO1, Rac1 |
NOx4 | P22PhOx |
NOx5 | |
DuOx1 | |
DuOx2 |
Membrane-bound cytochrome-B245 is a heterodimer formed by a 22-kDa polypeptide (P22PhOx, or light α chain encoded by the CYBA gene [cytochrome-B245 and -B558 (CyB) α-polypeptide]) and a 91-kDa glycoprotein (GP91PhOx, or heavy β chain encoded by the CYBB gene [CyB β-polypeptide]) and a component of NADPH oxidase, which synthesizes superoxide (O2 − ).
The catalytic component of cytochrome-B558 localizes to the membrane; it is constituted by the GP91PhOx–P22PhOx heterodimer (CyBβ–CyBβor CyBHC–CyBLC). Other regulatory components, such as P47PhOx and P67PhOx, as well as Rap1A and Rac regulators, reside in the cytoplasm.
10.5.2 Subtypes
A large number of potential combinations of subunits exists. Therefore, NADPH oxidases are categorized according to NOx constituents. NADPH oxidase isoforms are tissue specific.
NADPH oxidase isoforms NOx1 to NOx3 (Tables 10.14 to 10.16) are complexes with an activator P22PhOx subunit [1220]. However, NOx2 requires 2 additional activator subunits (P47PhOx and P67PhOx) in addition to P22PhOx to be active. Subunit P47PhOx can be phosphorylated by different kinases, such as PKCζ and P38MAPK. Regulatory subunits, such as NOxO1 and -2 and NOxA1 and -2, are components of different oxidase complexes.
Table 10.14.
Subtypes of NADPH oxidases (catalytic subunits and corresponding [possibly heteromeric] oxidase; Sources: [76, 1043]; KOx: kidney oxidase; LNOx: long (large) NOx; MOx: mitogenic oxidase; NOD: nucleotide-binding oligomerization domain-containing protein; NOH: NADPH oxidase homolog; NOxEF: NADH/NADPH oxidase/peroxidase EF hand domain-containing protein; ReNOx: renal NADPH-oxidase; ThOx: thyroid oxidase; TPO: thyroid peroxidase; TxnDC: thioredoxin domain-containing protein).
Type | Other aliases | Interactors |
---|---|---|
NOx1 | MOx1, NOH1, | NOxA1, CyBα/β, |
GP91-2, P65MOx | MAPK11, RelA | |
NOx2 | CyBβ, | CyBα, NOxA2, Rac1, |
GP91-1, GP91PhOx | IQGAP1, actin-γ1 | |
NOx3 | MOx2, | MAPK11–MAPK13, |
GP91-3 | MMP2/9 | |
NOx4 | KOx, KOx1, ReNOx | CyBα, |
TLR4, RelA, PTPn1 | ||
NOx5 | NOx5a/b | Abl |
DuOx1 | ThOx1, LNOx1, | TPO, TxnDC11 |
NOxEF1 | ||
DuOx2 | ThOx2, LNOx2, | NOD2, TxnDC11 |
NOxEF2 |
Table 10.15.
NADPH oxidase isoforms (Part 1) NOx proteins (Sources: [1218, 1221]). Component P22PhOx is essential for NOx activity; it complexes with other subunits to form NOx oxidases (NOx1–NOx3). In general, the organizer subunit of NOx1 and NOx3 is NOxO1, and that of NOx2 is NOxO2 (P47PhOx); the activator subunit for NOx1 is NOxA1 and that for NOX2 is NOxA2 (P67PhOx). Isotype NOx3 may not need an activator subunit. Isozyme NOx4 does not require cytosolic subunits. Production of P22PhOx subunit is controlled by AP1 and NFκB transcription factors.
Isoform | Distribution |
---|---|
Expression stimuli | |
Cell site | |
NOx1 | Vasculature, kidney, retina, |
colon, uterus, prostate, placenta | |
Smooth muscle and endothelial cells, pericytes, | |
osteoclasts | |
Upregulation by Infγ | |
Plasma membrane, caveolin | |
NOx2 | Vasculature, heart, lung, skeletal muscle |
Phagocytes, B lymphocytes, neurons, | |
endothelial and smooth muscle cells, cardiomyocytes, | |
hematopoietic stem cells, hepatocytes | |
Upregulation by Infγ | |
Intracellular | |
NOx3 | Fetal kidney, lung, liver, spleen, |
skull bone, brain, inner ear | |
Plasma membrane, vinculin | |
NOx4 | Vasculature, heart, kidney, skeletal muscle, |
bone, eye, ovary, placenta | |
Stimulation by ATn2, TGFβ, TNFα | |
Inhibition by BMP4 | |
Intracellular | |
NOx5 | Brain, vasculature, lymphoid tissue, |
testis, pancreas, stomach, prostate, | |
breast, placenta, ovary, uterus | |
Endothelium and smooth muscle cells |
Isoform | Distribution |
---|---|
Expression stimuli | |
Cell site | |
DuOx1 | Lung, thyroid, tongue, cerebellum, testis |
Upregulation by IL1, IL3, IL4 and Infγ | |
Plasma membrane | |
Calcium sensitive | |
DuOx2 | Gastrointestinal tract, salivary glands, thyroid, |
airway epithelia, uterus, gall bladder, pancreatic islets | |
Calcium sensitive |
Table 10.17.
NADPH oxidases in vascular cells (Source: [1225, 1228]; EC: endothelial cell; VSMC: vascular smooth muscle cell). Vascular NOx comprises 3 main catalytic subunits (NOx1, NOx2, NOx4) and 4 cytosolic regulatory components (NOxO1, NOxA1, Rac1, and Rac2). Endothelial cells possess NOx1, NOx2, NOx4, and NOx5, but only NOx2 and NOx4 abound. Isozyme NOx5 is extensively expressed in lymphatic cells. In vascular smooth muscle cells, NADPH oxidases can be activated by angiotensin-2, lysophosphatidylcholine, thrombin, and tumor-necrosis factor-α; redox-sensitive protein kinases include, in particular, JaK2, PKB, and P38MAPK.
Cell type | NOx subunit types |
---|---|
EC | P22PhOx, P47PhOx (NOxO2), Rac, |
NOx1/2/4/5 | |
VSMC | P22PhOx, P47PhOx, Rac, |
NOx1/2/4/5 | |
Fibroblast | P22PhOx, P47PhOx, Rac, |
NOx2/4 | |
Cardiomyocyte | NOx2/4 |
All members of the NOX family share common structural characteristics, such as 6 hydrophobic transmembrane domains, NADPH- and FAD-binding motifs in the cytoplasmic region, and 2 heme moieties in the intramembranous sequence.
In addition, NOx5 contains an N-terminal extension with 4 Ca
-binding EF hand domains [1222].88 Whereas NOx1, NOx2, and NOx3 require cytosolic subunits and cofactors for full activity, NOx5 can be activated by Ca
ion alone.


However, NADPH oxidases without EF hand domains associates with partners to sense and react to elevated intracellular Ca
concentration. For example, S100A8 and S100A9 proteins with 2 EF-hands that are expressed mainly in phagocytes heterodimerize upon increased Ca
concentration; this dimer then connects directly to the P22PhOx–GP91PhOx complex [1222].


In addition, NOx5 is activated by Abl kinase via a Ca
-mediated, redox-dependent signaling pathway [1223]. Therefore, a positive feedback occurs, as H2O2 can activate NOx5 via cytosolic protein Tyr kinase Abl.

Four NOx5 isoforms (NOx5α–NOx5δ) possess a long intracellular N-terminus that has Ca
-binding EF hand domains. They are produced in human aortic smooth muscle cells. A fifth NOx5 isoform (NOx5ε or NOX5S) lacks the EF-hand region, thereby resembling NOx1 to NOx4 isoforms [1218]. Unlike other NOx subtypes, NOx5 does need for its activity neither P22PhOx, nor cytosolic organizer or activator subunits, nor any cytosolic proteins.

NADPH oxidase-1 (NOx1) is a pyridine nucleotide-dependent, superoxide-generating oxidase homolog of the catalytic subunit of the NADPH oxidase of phagocytes (GP91PhOx). NADPH oxidase NOx1 contains regulatory cytosolic subunits P47PhOx (NOxO1), P67PhOx (NOxA2), and Rac1 GTPase. Phosphorylation by protein kinase-A of NoxA2 (Ser172 and Ser461) provokes its interaction with 14-3-3ζ protein and subsequent inhibition of NOx1 activity.
Subtype NOx2 possesses several regulatory components (P22PhOx, P4OPhOx, P47PhOx, P67PhOx, and Rac1 GTPase), in addition to NOx2 catalytic subunit. In other words, NOx2 oxidase, a heterodimer made up of NOx2 subunit (i.e., GP91PhOx or CyBβ) and P22PhOx, is activated when the P22PhOx–GP91PhOx heterodimer associates with P40PhOx, P47PhOx (NOxO2), P67PhOx (NOxA2), and Rac1 or Rac2 GTPase.
Unlike NOx1 and NOx2, NOx4 activation does not require P47PhOx, P67PhOx, or Rac GTPase.
At least 4 NOx homologs reside in vascular cells (Table 10.17). NADPH oxidase-1 is expressed in vascular smooth muscle cells, particularly upon stimulation by platelet-derived growth factor, but not in blood leukocytes. In vascular smooth muscle cells, prostaglandin F2α and angiotensin-2 also provoke NOx1 production. Subtype NOx1 serves asvoltage-gated proton (hydrogen) channels involved in cell defense against acidic stress [1224].
Whereas NOx1 and NOx2 that lodge mainly in the plasma membrane generate O2 − , NOx4 that resides in the endoplasmic reticulum and focal adhesions produces primarily H2O2 agent. Isotype NOx1 colocalizes with caveolin in patches of the plasma membrane, whereas NOx4 resides with vinculin in focal adhesions [1226]. Endothelial cells and adventitial fibroblasts synthesize mainly NOx2 and NOx4 and vascular smooth muscle cells NOx1, NOx2, and NOx4 isozymes [1225].
Vascular NOx5 is implicated in: (1) endothelial cell proliferation and angiogenesis; (2) PDGF-induced proliferation of vascular smooth muscle cells; and (3) atherosclerosis [1225]. Vascular NOx5 is activated by thrombin and platelet-derived growth factor.
In vascular smooth muscle cells, reactive oxygen species act as signaling molecules that contribute to cell growth, hypertrophy, and migration. Rodent aortic smooth muscle cells mainly possess NOx1 and NOx4 subtypes; NOx2 is expressed at very low levels. Whereas NOx1 is inactive under basal conditions, NOx4 is constitutively active. In human aortic smooth muscle cells, synthesis of NOx1 and NOx4 isoforms as well as P22PhOx, P47PhOx, and P67PhOx subunits is regulated by the JaK–STAT signaling cascade, especially STAT1 and STAT3 transcription factors [1227].
In endothelial cells, NOx2 and NOx4 are predominantly ( ∼ 90%) located in the endoplasmic reticulum and nuclear membranes.
Members of the DUOX set possess a NOx1–NOx4 homology domain, an EF-hand region, and a seventh transmembrane domain at the N-terminus with an ecto-facing peroxidase like domain [1218].89 These enzymes are glycosylated. Immature, partly glycosylated form of DuOx2 generates superoxide, whereas mature form produces hydrogen peroxide [1218]. The DuOx enzymes do not require activator or organizer subunits. Enzyme DuOx1 is synthesized in response to interleukin-4 and -13 and DuOx2 to interferon-γ in respiratory epithelia [1218].
10.5.3 Activity
NADPH oxidases have constitutive and inducible activities as well as substrate specificity. Stimulation leads to subunit assembling. Activated neutrophils, monocytes, and macrophages produce large quantities of superoxide for host defense via NOx2 enzyme. Fibroblasts, vascular smooth muscle and endothelial cells, and cardiomyocytes contain several NADPH oxidase family members to regulate signaling cascades. Different NOx isozymes activate different kinases: (1) in NOx2-overexpressing cells, P38MAPKs are phosphorylated in response to angiotensin-2; (2) in NOx4-overexpressing cells, ERK1, ERK2, and JNKs are activated [1225].
NADPH oxidases interact with small Rac1 or Rac2 GTPases, according to the cell type. Subtypes NOx1 and NOx2 increase Rac GTPase activity.
Isoforms NOx1 and NOx2 are directly and indirectly activated by TNFα, respectively [1220]. Vascular NADPH oxidases are activated by protein kinase-C. Phospholipase-A2 and -D may also stimulate NOx via effectors. Thrombin, several growth factors, and angiotensin-2 stimulate NOx activity in vascular smooth muscle and endothelial cells (Table 10.18). Besides, angiotensin-2 transactivates EGF receptor with ROS via Src kinase.
Table 10.18.
Expression of NAD(P)H oxidase in vascular smooth muscle cells depends on numerous compounds that either favor or prevent its activity.
Upregulation | Downregulation |
---|---|
ATn2 | Leptin |
ET1 | Adiponectin |
Thrombin | Estradiol |
PDGF | Statins |
TGFβ | |
TNFα | |
PGF2α | |
oxLDL | |
WSS | |
Fatty acids |
Isozymes NOx2 and NOx4 that are coexpressed in many cell types respond distinctively to stimulations by angiotensin-2, TNFα, and insulin. They regulate the activation of different protein kinases. In particular, insulin raises phosphorylation of P38MAPK, PKB, and GSK3β specifically in NOx4-overexpressing cells and JNK specifically in NOx2-overexpressing cells [1229]. In addition, activation caused by angiotensin-2 of ERK1, ERK2, and P38MAPK depends on NOx2 isoform.
NADPH oxidase is able to sense mechanical stress. It can particularly react to directional changes of flow. Activated NOx generates superoxide (O2 − ) in a dose-dependent fashion during flow reversal [1230]. Superoxide can eliminate nitric oxide and provoke endothelial dysfunction.
Vascular NOxs are regulated via synthesis and post-translational modifications such as phosphorylation by mechanical stresses, chemical agents (pH, O2), and vasoactive factors (angiotensin-2, endothelin-1, and aldosterone) and growth factors. In endothelial cells, NOx5 is activated by vasoactive peptides angiotensin-2 and endothelin-1 via a Ca
–calmodulin-dependent, Rac1-independent mechanisms. Subsequently generated ROS lead to ERK1/2 phosphorylation under stimulation by angiotensin-2, but not endothelin-1 [1225].

In hyperglycemia, NADPH oxidase excessively generates reactive oxygen species that impair anti-oxidant defense, especially redox-sensitive transcription factors involved in adaptive responses to oxidative stress [1221].90 Whereas NADPH contributes to the anti-oxidant potential by regenerating reduced glutathione from oxidized glutathione, high glucose concentration (10–25 mmol/l) activates protein kinase-A that phosphorylates (inhibits) glucose-6 phosphate dehydrogenase, thereby leading to decreased NADPH level.91 Therefore, sustained NOx activation in diabetes reduces intracellular concentration of NADPH that is a cofactor for NOS3 and several anti-oxidant enzymes. Prolonged NOx excitation thus causes oxidative stress with NOS3 uncoupling,92 mitochondrial dysfunction, attenuated anti-oxidant gene expression, and eventually endothelial dysfunction (Table 10.19). However, NADPH oxidase can provoke a transient anti-oxidant response via receptor Tyr kinases and redox-sensitive transcription factors, such as NF-E2-related factor NRF2 [1221].93
Basal activity | Sustained stimulation |
---|---|
Anti-oxidant gene expression | ROS overproduction |
Oxygen sensing (HIF1α) | Mitochondrial dysfunction |
NOS3 activity | NOS3 uncoupling |
10.5.4 NOx–ROS Axis
In vascular cells, NADPH oxidase isoforms — NOx1, NOx2, NOx4, and NOx5 — differ in their response to stimuli, activity, and released ROS type [1231]. In particular, NOx activator NoxA1 regulates redox signaling and VSMC phenotype [1232]. Subtype NOx1 is involved in responses to stimuli of vascular smooth muscle cells (hypertrophy upon angiotensin-2 stimulation, serum-induced proliferation, and migration primed by fibroblast growth factor-2).
In cultured smooth muscle cells from thoracic aorta, NADPH oxidase operates via Src-mediated phosphorylation (transactivation) of epidermal growth factor receptor to activate matrix metallopeptidase MMP9 and promote cell migration [1233].94 Alteration in N-cadherin-mediated intercellular adhesion that results from shedding of the extracellular portion of N-cadherin by MMP9 enables VSMC migration from the media to the subendothelial space, where they can proliferate. This migration that relies on elevation of β-catenin signaling and cyclin-D1 production support intimal thickening [1231].
In atherosclerosis, heightened thrombin synthesis and activity raise NOx activity and then ROS production [1233].
Migration of vascular smooth muscle cells that depends on NOx1 relies on JNK and the Src–PDK1–PAK axis under stimulation by FGF2 and PDGF, respectively [1231]. On the other hand, NOx1-dependent, thrombin-induced VSMC migration depends on P38MAPK activation upon ROS stimulation.
10.6 Redox Signaling and Reactive Oxygen and Nitrogen Species
During redox signaling, free radicals (atoms, molecules, or ions with unpaired electrons), reactive oxygen species, and other electronically activated species act as intra- and extracellular messengers (Table 10.20). Reactive oxygen species modify subtrates by oxidizing thiol groups (-SH),95 thereby forming disulfide bonds (-S–S-)96 that reversibly influence protein structure and function:
-SH + -SH
-S–S- + 2 H + + 2 e − .

Table 10.20.
Major components of the redox signaling pathway (Source: [10]; ROS: reactive oxygen species). Free radicals are implicated in cell signaling and phagocytosis, but their accumulation causes oxidative stress, damages DNA, proteins, carbohydrates, and lipids, thereby provoking cell injury. Consequently, both enzymes that synthesize and catabolize free radicals are required for normal cell function and minimization of induced damage. Sulfiredoxin catalyzes the reduction of hyperperoxidized proteins. Vitamin-A, -C, and -E, bilirubin, uric acid, dietary polyphenol anti-oxidants help neutralize free radicals.
Component | Members |
---|---|
ROS | Superoxide (O2 ∙ − ) |
Hydrogen peroxide (H2O2) | |
Hydroxyl radical (OH ∙ ) | |
Enzymes of the ROS synthesis | NADPH oxidases |
Dual oxidases | |
Enzymes of the ROS metabolism | Superoxide dismutase |
Catalase (peroxisome) | |
Glutathione peroxidase (cytosol | |
and mitochondria) | |
Peroxiredoxins | |
Thiol-containing proteins | Glutathione |
Glutaredoxin | |
Thioredoxin | |
Reductases | Glutathione reductase |
Glutaredoxin reductase | |
Thioredoxin reductase | |
Sulfiredoxin |
Target proteins with reduction–oxidation (redox) sensitive thiol groups include: (1) signaling mediators, such as mitogen-activated protein kinases and protein Tyr phosphatases [1234]; (2) transcription factors; (3) RNA-binding proteins used in DNA methylation; and (4) mediators of histone acetylation, deacetylation, or methylation [1235]. Protein S-glutathiolation and disulfide formation contribute to the redox regulation of signaling proteins and protein regulation during intracellular oxidative stress.
Redox signaling participates in many cellular processes: gene transcription,97 modulation of Ca
signaling,98 DNA damage, cell proliferation,99 apoptosis,100 and vascular tone [10].101

The intracellular medium is a reducing environment. The cell has a large redox buffer capacity using glutathione, which maintains the intracellular redox balance. The redox system signals as well as modulates the activity of other signaling pathways. Conversely, Ca
can stimulate redox signaling, particularly in mitochondria. On the other hand, oxidative stress can cause apoptosis.

The redox balance of the cell is maintained by the energy metabolism, primarily the pentose phosphate pathway that creates NADPH. The latter maintains redox buffers such as glutathione.
The pentose phosphate pathway, also called the pentose phosphate shunt, the phosphogluconate pathway, and the hexose monophosphate shunt, generates NADPH and pentoses (5-carbon carbohydrates).102
Non-phagocytic cells use reactive oxygen species (ROS) as intracellular messengers to activate signaling cascades. On the other hand, phagocytes such as neutrophils rapidly generate superoxide radical (O2 − ) and hydrogen peroxide (H2O2) that can be released in the extracellular space to kill invading microorganisms and cells.
Free radicals and anti-oxidants are involved in cell signaling and activation or inhibition of transcription factors. In addition, the synthesis of growth factors and cytokines, such as platelet-derived (PDGF) and epidermal (EGF) growth factor, transforming growth factor-β, and tumor-necrosis factor-α, may be influenced by free radicals.
Two main types of redox signaling exist: signaling mediated by reactive oxygen species and that primed by reactive nitrogen species (RNS), associated with the NO–cGMP pathway.
10.6.1 Signaling by Reactive Oxygen Species
Reactive oxygen species can operate as second messengers produced at appropriate concentrations by various enzymes, such as nicotinamide adenine dinucleotide phosphate (NADPH) oxidase (NOx) and Dual oxidase (DuOx; Sect. 10.5), especially for cell proliferation. At abnormal concentrations, they function as noxious molecules that target various cellular components, thereby causing oxidative damage and thus aging, cell death, and human diseases.
Reactive oxygen species refer to oxygen species — superoxide, hydrogen peroxide, and hydroxyl radical — that are more reactive than oxygen. Several proteins, such as protein Tyr phosphatases and members of the thioredoxin (TRX) and peroxiredoxin (PRX) families, that commonly possess a highly reactive cysteine residue function as ROS effectors; they are reversibly oxidized by ROS agents.
Oxygen is a strong oxidizing agent that possesses 2 unpaired electrons (one by oxygen atom), which have parallel spins and occupy separate π-antibonding orbitals. These unpaired electrons confer to oxygen the feature of a free radical. However, oxygen is relatively inert, because it has to accept a pair of electrons with antiparallel spins to fit into the empty spaces in the π orbitals to react with another molecule. Oxygen accepts electrons sequentially, thereby forming successively different ROS agents. Superoxide (O2 ∙ − [ ∙ : radical] or simply O2 − ) is transformed by the addition of electrons into hydrogen peroxide (H2O2). The generation of hydroxyl radical (OH ∙ ) then follows. Therefore, ROS are not all free radicals, as free radicals are atoms and molecules with at least one unpaired electrons.
Cells of respiratory epithelia are exposed to higher oxygen concentrations than most other tissues, hence with a greater probability to free radicals. Rapid catabolism of reactive oxygen species ensures a short half-life.
The sequential ROS synthesis can be triggered by an external messenger that activates its cognate receptors to generate superoxide radical. Numerous receptors activated by external messengers stimulate the formation of reactive oxygen species, such as receptors of cytokines (tumor-necrosis factor-α, interleukin-1, and interferon-γ), receptor Tyr kinases of growth factors (platelet-derived, epidermal, and fibroblast growth factor) and G-protein-coupled receptors of various regulators (angiotensin-2, bradykinin, endothelin, serotonin, and thrombin). The above-mentioned regulators activate receptors coupled to PI3K, thereby synthesizing PIP3 that then stimulates plasmalemmal NADPH oxidases via Rac GTPase. NADPH oxidase removes an electron from NADPH and transfers it to oxygen to create superoxide radical.
In addition to the plasma membrane, mitochondria also generate reactive oxygen species. In mitochondria, most electrons that enter the electron transport chain are transferred to oxygen, oxygen being reduced to water by accepting 4 electrons from cytochrome-C oxidase:
O2 + 4 e − + 4 H +
ions to stimulate the formation of the mitochondrial permeability transition pore.

Oxidized proteins can prime gene transcription, in addition to the modulation of the activity of ion channels, in particular of the Ca
flux, and activated mitogen-activated protein kinase modules.

Signaling molecules, the activity of which is reduced by oxidation, include protein Tyr phosphatases and PTen phosphatase, a protein TyrP/SerP/ThrP phosphatase that preferentially dephosphorylates phosphoinositides. Other proteins that possess cysteine residues hypersensitive to oxidation (Cys
) include the cell cycle regulatory phosphatase CDC25c and Ca
-release channels, ryanodine receptors and inositol trisphosphate receptors.


10.6.1.1 Superoxide
The superoxide radical (O2 − ) that results from the one-electron reduction of O2 is short-lived (half-life
[1 μs]) due to the rapid transformation by superoxide dismutate (SOD) into hydrogen peroxide. Superoxide anion is rapidly converted by adding further electrons into hydrogen peroxide by superoxide dismutase. Hydrogen peroxide, one of the main messengers used by the redox signaling pathway, provokes oxidation of target proteins.

10.6.1.2 Superoxide Dismutases
The most important anti-oxidant enzymes that scavenge superoxide are intra- and extracellular (SOD ec ) superoxide dismutases. Superoxide dismutases (SOD) constitute a family of metallopeptidases that converts superoxide radical into hydrogen peroxide:
2 O2 − + 2 H + → H2O2 + O2.
Subtypes of superoxide dismutases encompass: (1) iron-containing superoxide dismutases (FeSOD); (2) copper-containing superoxide dismutases (CuSOD); (3) copper and zinc-containing superoxide dismutases ((Cu,Zn)SOD); and (4) manganese-containing superoxide dismutases (MnSOD).
In the lung, the MnSOD subtype is weakly expressed in alveolar macrophages and airway epithelial cells. It is induced by cytokines [1237]. (Cu,Zn)SOD is constitutively expressed in human bronchial and alveolar epithelium. Extracellular SOD ec is mainly released from alveolar macrophages, in regions that contain high amounts of collagen-1 fibers and near lung capillaries.
10.6.1.3 Hydrogen Peroxide
Like superoxide (O2 − ), hydrogen peroxide (H2O2) has a short half-life due to its rapid catabolism. Its action depends upon its ability to react with cysteine residues of target proteins that have reactive thiol groups, which are rapidly oxidized to form a disulfide bond. Conversely, glutaredoxin and/or thioredoxin allow the recovery from the oxidized state back to a fully reduced thiol group.
Upon receptor activation and subsequent stimulation of NADPH oxidase, H2O2 is generated near the plasma membrane, once PI(3,4,5)P3 is synthesized. Hydrogen peroxide inhibits phosphatase and tensin homolog deleted on chromosome 10 (PTen) that hydrolyzes PIP3, thereby initiating a positive feedback loop.
Oxidation utilizes numerous modes, but is specific, as H2O2 selectively modifies only a subset of proteins. Proteins can indeed be characterized by their sensitivity to mild oxidizing agents such as H2O2. Most of the cysteine residues in proteins resists to oxidation by H2O2 agent. However, some of the cysteine residues, particularly those located next to positively charged amino acids, exist as thiolate anions (Cys
) that are very vulnerable to oxidation (hyperreactive cysteine residues).

Hydrogen peroxide targets, in particular, the reduced cysteine residues in N-termini (NCysSH) of thioredoxin dimers to create 2 oxidized sulfenic cysteine residues (NCysSOH). These residues then interact with CCysSH of C-termini of neighboring dimers to form 2 intermolecular disulfide bonds, structural units composed of a linked pair of sulfur atoms. Hydrogen peroxide is transformed by a set of enzymes: catalase, glutathione peroxidase (GPx), and peroxiredoxin (Prx).
In fact, the unstable sulfenic acid group (-SOH) can be processed using different modes [10]. (1) The sulfenic acid residue can be converted into an intramolecular disulfide bond with the elimination of water. (2) The sulfenic acid residue can interact with the reduced form of glutathione (GSH) to form an intermolecular disulfide bond. (3) The sulfenic acid residue can interact with the main-chain nitrogen atom of an adjacent serine residue to form an intramolecular cyclic sulfenyl amide (-SN), as during the oxidation of protein Tyr phosphatases (e.g., PTPn1). (4) The sulfenic acid residue can undergo hyperperoxidation by interacting with another molecule of H2O2 to form a sulfinic acid intermediate (-SO2H; CysSO 2H). (5) The sulfinic acid intermediate undergoes further hyperperoxidation to form the sulfonic acid intermediate (-SO3H; CysSO 3H). (6) The sulfinic acid group can be reduced by sulfiredoxin with ATP.
The irreversible oxidation may be avoided by an internal reaction. The sulfenic acid is rapidly converted into a sulfenyl-amide species, as it reacts with the neighboring amide nitrogen to yield a cyclic sulfenyl amide (Cys–S–N). This sulfenyl-amide intermediate protects against further oxidation, and, upon enzyme reactivation, can be converted back into a thiol group.103
Hydrogen peroxide can be catabolized by 3 main types of enzymes: catalase, glutathione peroxidase, and peroxiredoxins.
10.6.1.4 Catalase
Heme-containing catalase decomposes hydrogen peroxide to water and oxygen:
2 H2O2 → 2 H2 + O2.
Most of the catalases in the cell lodge in peroxisomes. Their action is thus restricted to H2O2 produced at the plasma membrane.
10.6.1.5 Glutathione Redox Couple (GSH–GSSG)
Glutathione is a Glu–CysH–Gly tripeptide. Two reduced forms of GSH molecules tether together via a disulfide bond to create the oxidized form, glutathione disulfide (GSSG). The 2GSH–GSSG redox couple is the most abundant redox couple in the cell (cytoplasmic GSH concentration 1–10 mmol) [10]. The oxidized form of glutathione (GSSG) is converted back into GSH by glutathione reductase.
The oxidized form of glutathione interacts with reduced glutaredoxin (GRx(SH) 2 to generate oxidized glutaredoxin (GRxS 2); this disulfide bond can be transferred to oxidize target proteins.
Under normal reducing conditions, the cell reduction potential is high and cell proliferation is favored. Cell differentiation seems to occur at lower potentials. Low potentials supports apoptosis [10].
10.6.1.6 Glutathione Reductase
Glutathione reductase converts oxidized form of glutathione (GSSG) back into the reduced form (GSH) [10]:
GSSG + NADPH + H + → 2 GSH + NADP + .
10.6.1.7 Glutathione Peroxidase
Glutathione peroxidases (GPx) use the reducing capacity of glutathione to convert H2O2 into water:
H2O2 + 2 GSH
2 H2O + GSSG.

Human bronchial epithelial cells, alveolar type-2 cells, and macrophages are characterized by a constitutive activity of catalase and glutathione peroxidase. The epithelial lining fluid of airways has a glutathione level more than 100 times higher than that in circulating blood.
10.6.1.8 Peroxiredoxins
Peroxiredoxins (PRx) are thiol-specific anti-oxidant enzymes that catabolize hydrogen peroxide into water:
H2O2 + PRx(SH) 2
2 H2O + PRxS 2.

They also exert a protective role using their peroxidase activity against peroxynitrite and phospholipid hydroperoxides. They homo-oligomerize under strong oxidative stress.
The active cysteine site of peroxiredoxins can be selectively oxidized to cysteine sulfinic acid (CysSO(OH)), thereby inactivating the peroxidase activity. Nonetheless, the sulfinic form of peroxiredoxin-1 produced during the cell exposure to H2O2 is rapidly reduced to the catalytically active thiol form [1236].
The PRX family contains 6 ubiquitous members, especially in human lung cells [1237] (Tables 10.21 and 10.22). These proteins are classified into 3 subfamilies: 2-cysteine (2−CysPrx), atypical 2−CysPrx, and 1−CysPrx according to the number and position of cysteine residues that participate in the catalytic activity.
Isoform | Features |
---|---|
2−CysPrx | |
PRx1 | Cytosol, inducible, 22 kDa |
PRx2 | Cytosol, inducible, 22 kDa |
PRx3 | Mitochondria, 28 kDa |
PRx4 | Endoplasmic reticulum, 31 kDa, |
extracellular space (heparin binding) | |
PRx5 | Atypical 2−CysPrx, 17 kDa, |
cytosol, peroxisomes, mitochondria | |
PRx6 | 1−CysPrx, 25 kDa |
Table 10.22.
Distribution and expression degree (weak + , mid
and strong
) of peroxiredoxins in the normal human lung (Source: [1237]).


Isoform | Bronchial | Alveolar | Alveolar | Vascular |
---|---|---|---|---|
epithelium | epithelium | macrophages | endothelial cells | |
PRx1 | ![]() | + | ![]() | + |
PRx2 | + | + | + | |
PRx3 | ![]() | ![]() | ![]() | + |
PRx4 | + | + | ||
PRx5 | ![]() | ![]() | ![]() | |
PRx6 | ![]() | ![]() | ![]() | + |
Peroxiredoxin-6 is detected in bronchial Clara cells, alveolar epithelial type-2 cells, and alveolar macrophages [1238]. It regulates cellular signaling and protects against membrane lipid peroxidation and apoptosis caused by oxidative stress. It can indeed reduce phospholipid hydroperoxides, thereby being capable of repairing membrane damage caused by oxidative stress.
Peroxiredoxins contain catalytic cysteine residues. They use thioredoxin as an electron donor. The reducing equivalents derived from thioredoxin (Trx) are used to regenerate PrxSH 2:
PrxS 2 + TrxSH 2 → PrxSH 2 + TrxS 2.
10.6.1.9 Glutaredoxin
Recovery from oxidation is based on thioredoxin (TRx) and glutaredoxin (GRx). These 2 proteins have common and distinct features. In particular, they are characterized by their substrate specificity and types of target disulfide bonds. For example, GRx is a less efficient reducing agent on protein Tyr phosphatase PTP1b than TRx agent.
In a reduced state, both TRx and GRx reduce their substrates and become oxidized. Oxidized glutaredoxin is converts back into a reduced state using glutathione, which is regenerated by a glutathione reductase.
10.6.1.10 Thioredoxin
The various oxidated intermediates can be converted back into the initial reduced state by either thioredoxin or glutaredoxin. In particular, the anti-oxidant defense of the human lung include both small cysteine-containing proteins thioredoxin and peroxiredoxins.
Ubiquitous thioredoxins act as anti-oxidants that foster the reduction of proteins by cysteine thiol–disulfide exchange. The redox regulator thioredoxin possess multiple substrates, such as ribonuclease, chorionic gonadotropin (a glycoprotein hormone produced during pregnancy), coagulation factors, glucocorticoid receptor, and insulin [10].
The reducing ability of the regulator thioredoxin is repressed by Ca
ions that support the oxidized form of reduced thioredoxin (TRxS 2) [10].

Reduced TRx(SH) 2 bind to MAP3K5 enzyme.104 When it is oxidized (TRxS 2), MAP3K5 is released and causes apoptosis [10].
Thioredoxin may also increase the expression of hypoxia-inducible factor HIF1α, thereby supporting the activity of vascular endothelial growth factor and angiogenesis [10].
10.6.1.11 Thioredoxin Reductase
Thioredoxin reductase converts back oxidized thioredoxin into a reduced state. Hence, thioredoxin reductase and thioredoxin compose an important redox regulator. Three proteins constitute the TRX family: TRx1, mitochondria-specific TRx2, and sperm spTRx. Thioredoxin-2 regulate the mitochondrial membrane potential and contributes to the inhibition of apoptosis.
Thioredoxin reductase contains selenocysteine (SeCys) in its C-terminal active site as well as flavin adenine dinucleotide (FAD) in its N-terminal region. This enzyme operates by transferring electrons from NADPH to FAD and then on to the C-terminal active site.
The thioredoxin redox regulator controls numerous transcription factors involved in cell proliferation and death. In human adipose tissue-derived mesenchymal stem cells, overexpression of TRx1 and TRx2 increases ERK1 and ERK2 phosphorylation, nuclear factor-κB activation, and βCtn–TCF–promoter activity, but prevents leucine zipper tumor suppressor LZTS2 expression [1239].
10.6.1.12 Nucleoredoxin
Nucleoredoxin (NRx) that mainly localizes to the nucleus, but also in the cytoplasm. This oxidoreductase105 contains a pair of Cys residues in its catalytic sequence. It inhibits Disheveled, thereby suppressing Wnt signaling. However, NRx impedes Dvl ubiquitination and subsequent degradation, hence maintaining a pool of inactive Dvl for robust signaling under Wnt stimulation.
10.6.1.13 Peroxidases
Peroxidases catalyze the oxidation of substrates by hydrogen peroxide. Thyroid peroxidase (TPO) are used in the oxidation of iodine and formation of thyroid hormone. Myeloperoxidase (MPO) of neutrophils, monocytes, and macrophages serve in the production of the weak acid and bactericidal oxidizer hypochlorous acid (HOCl) from hydrogen peroxide (H2O2) and chloride anion (Cl − ).106 Hypochlorous acid reacts with various types of molecules, such as DNA, RNA, fatty acids, cholesterol, and proteins.
The set of peroxidases also includes lactoperoxidase, eosinophil peroxidase, and salivary peroxidase, as well as vascular peroxidase (VPO1; or insect peroxidasin homolog) that have a very low enzymatic activity in comparison with other peroxidases [1240].
Heme-containing VPO1 can be produced and secreted into the extracellular medium upon stimulation by transforming growth factor-β1 [1240]. It is mainly detected in the vascular wall, lung, liver, among other organs [1241].
Increased synthesis of reactive oxygen species can foster smooth muscle cell proliferation and hypertrophy as well as matrix formation. In the cardiovascular system, myeloperoxidase promotes lipid peroxidation and scavenges nitric oxide. Its products activate matrix metallopeptidases, thereby favoring tissue remodeling. In addition, vascular peroxidase contributes to the proliferation of vascular smooth muscle cells caused by angiotensin-2 via the NOx-H2O2–VPO1–HOCl–ERK1/2 pathway [1241]. Hypochlorous acid is synthesized upon angiotensin-2 stimulation. Angiotensin-2, like another profibrotic agent TGFβ, activates NADPH oxidase that increases VPO1 expression. Vascular peroxidase-1 uses H2O2 produced by NADPH oxidase to generate peroxides such as hypochlorous acid. The latter modifies intracellular proteins and, once it is secreted, matrix proteins.
10.6.1.14 Hydroxyl Radical
Hydroxyl radical (OH ∙ ) is the highly reactive, short-lived (half life
[1 ns]), neutral form of the hydroxide ion [(OH) − ]. Hydrogen peroxide can be converted into highly toxic hydroxyl radical, as it undergoes a reduction catalyzed by transition metals ( Fe3 + or Cu
).


10.6.1.15 Cysteine Oxidation by Reactive Oxygen Species
Among 20 amino acids that build proteins, cysteine has a thiol moiety (R–SH functional group, R denoting an attached hydrogen, a hydrocarbon side chain, or any group of atoms) in the side chain of Cys is very sensitive to oxidation. This thiol moiety can form disulfide bonds with another thiol moiety (R–S–S–R) [1242]. These disulfide bonds can be degraded to reform free thiol moiety.
The thiol moiety (R–SH) is oxidized to become the sulfenyl moiety (R–SOH). This process is reversible, as the sulfenyl moiety can be reduced to the thiol moiety by various anti-oxidants. The sulfenyl moiety can form reversible disulfide bond with another thiol moiety. Disulfide bond can be reduced by various anti-oxidants. In turn, the sulfenyl moiety can be further oxidized to the sulfinyl (R–SO2H) and sulfonyl moiety (R–SO3H) that cannot be reduced under normal intracellular conditions.
Protein Tyr phosphatases possess a Cys residue in the catalytic domain that acts as a transient acceptor for the phosphate during dephosphorylation. Several PTPs are inhibited by oxidation of their catalytic Cys residue [1218]. This process can be observed in endothelial cells stimulated with PDGF factor [1242]. Phosphatase and tensin homolog deleted on chromosome 10 is a PTP domain-containing protein that can experience oxidation; the catalytic Cys residue forms a disulfide bond with another Cys that protects itself from further irreversible oxidation. Protein Tyr phosphatase PTPn1 contributes to the regulation of insulin signaling. Its oxidized Cys in the catalytic region forms a reversible sulfenyl-amide bond (R–S–N–R) with nitrogen atom in the polypeptidic chain that also protects it from further oxidation [1242]. Oxidation of MAPK phosphatases (MKP) prevents their phosphatase activity. Oxidized MKPs form proteic complexes labeled for proteasomal degradation.
On the other hand, Lyn kinase in leukocytes is activated by wound-derived H2O2 and triggers movement of leukocytes to wound sites [1242]. Ataxia-telangiectasia mutated protein kinase can be oxidized (activated) under oxidative stress, even in the absence of DNA double-strand breaks [427]. The oxidized ATMK form is a disulfide-crosslinked dimer.
Pyruvate kinase muscle isozyme PKM2 normally highly expressed only in undifferentiated tissues catalyzes the transfer of a high-energy phosphate group from phosphoenol pyruvate to ATP, i.e., the rate-limiting step of glycolysis.107 Oxidative stress causes PKM2 oxidation (Cys358), thereby provoking reversible enzyme inactivation [1245]. The diversion of glucose flux into the pentose phosphate pathway enables to generate sufficient reducing potential for ROS detoxification.
10.6.1.16 Summary of ROS Effects
10.6.1.17 Inhibition of Phosphatases and Activation of Kinases
Reactive oxygen species inhibit protein Tyr phosphatases that control cell proliferation, differentiation, survival, metabolism, and motility. On the other hand, they activate MAPK modules.
10.6.1.18 Regulation of Ion Channels
Reactive oxygen species can regulate intracellular and plasmalemmal ion channels (e.g., K + channels [inactivation], especially in the aging brain,CaV1 [activation], store-operated Ca
channels [inhibition],Ca
ATPases [activation at low ROS concentrations; inhibition during oxidative stress], and ryanodine receptors [stimulation]) directly or via effectors [1218]. Reactive oxygen species can regulate ion channel activity via either post-translational modifications (cysteine oxidation and S-glutathiolation) or cell depolarization that results from NOx-dependent electron transport.


protein kinase-G phosphorylates (inactivates) cardiacATP-sensitive, hetero-octamericpotassium channel (KATP,108 more precisely cardiac KIR6.2–SUR2a carrier used in metabolic stress adaptation in the heart, or some closely associated proteins. Kinase PKG also stimulates KATP channel via ROS (H2O2) in particular) and the calmodulin–CamK2 axis [1246].
Gene Expression
Generation of ROS stimulates the production of angiotensin-2, TNFα, TGFβ1, CCL2 chemokine, and plasminogen activator inhibitor-1 [1218]. Transcriptional upregulation results either from redox-sensitive second messengers, such as MAPK, or transcription factors (AP1, P53, and NFκB) with redox-sensitive cysteine residues in their DNA-binding domain.
Oxygen Sensing
Some organs, such as the kidney cortex, carotid bodies, and pulmonary hypoxia-sensitive neuroepithelial bodies, are specialized in oxygen sensing. Hypoxia may lower concentrations of by-products of O2 reduction by NADPH oxidase (ROS), thereby altering the cellular redox potential and priming a conformational change and inactivation of the redox-sensitive K + channels. The resulting drop in outward flux of K + ions leads to membrane depolarization, calcium influx, and neurotransmitter secretion. In addition, increased ROS generation under hypoxia can also contribute to HIF stabilization [1218].
Regulation of Matrix Metallopeptidases
Expression and/or activation of matrix metallopeptidases may be, at least partly, regulated by ROS agents.
Cell Fate
Under some circumstances, ROS may support cell proliferation. Agents ROS can accelerate cell senescence due to oxidative stress. Cell apoptosis can follow damage of DNA, lipids, and proteins or be primed by activated signaling mediators (JNKs, ERKs, and P38MAPKs) [1218]. Under certain circumstances, ROS may act as anti-apoptotic signals via NFκB and the PKB–MAP3K5 pathway.
10.6.2 Signaling by Reactive Nitrogen Species
Reactive nitrogen species (RNS) are antimicrobial molecules derived from free radical nitric oxide (NO ∙ ) and another free radical, superoxide (O2 ∙ − ), produced by inducible nitric oxide synthase NOS2 and NADPH oxidase, respectively. The former enzyme is expressed primarily in macrophages after stimulation by cytokines (e.g., interferon-γ) and microbial products (e.g., lipopolysaccharides). Reactive nitrogen species act synergistically with reactive oxygen species to cause nitrosative stress and damage their targets.
However, neither nitric oxide nor superoxide is particularly toxic when these substances do not accumulate. High concentrations of superoxide are avoided by superoxide dismutases located in mitochondria and cytosol, as well as in the extracellular matrix. Nitric oxide, after a quick intratissular transfer, is rapidly eliminated by erythrocytes, where it is rapidly converted to nitrate, as it reacts with oxyhemoglobin.109
The production of reactive nitrogen species begins with the very rapid reaction of free radical nitric oxide with superoxide, when they are synthesized simultaneously close to each other (but not necessarily in the same cell) to form peroxynitrite (ONOO − ), a strong oxidant:
NO ∙ + (O2 − ∙ → ONOO −
Peroxynitrite is able to cross cell membranes, especially that of erythrocytes, either in the anionic form through anion channel or in the protonated form by passive diffusion [1248]. It then rapidly reacts with oxyhemoglobin to yield methemoglobin.
Peroxynitrite can react with other molecules to form additional types of reactive nitrogen species, such as nitrogen dioxide (NO2 ∙ ) and dinitrogen trioxide (N2O3), as well as other types of free radicals (Table 10.23):
NO ∙ + NO2 ∙ ⇌ N2O3;
ONOO − + H + → ONOOH (peroxynitrous acid) → NO2 ∙ + OH ∙ ;
ONOO − + CO2 → ONOOCO2 − (nitrosoperoxycarbonate) → NO2 ∙ + O=C(O ∙ )O − (carbonate radical).
Table 10.23.
Relative contributions of oxidation, nitrosation, and nitration to products of reactive nitrogen species for a steady-state concentration [ ∙ NO] SS = 20 nmol (Source: [1249]). Reactions occur in an aqueous solution within the complex environment of the cell; some reaction types dominate because of competition for reactants and intermediates. The dominant reactions in a set of interacting rapid reactions are those involving reactants with the highest concentrations. Nitration introductes a nitro group (-NO2) into a chemical compound. Nitrosylation adds a nitrosyl ion (NO − ) to a metal or a thiol. Nitrosation connects a nitrosonium ion (NO + ) to an amine (-NH2) leading to a nitrosamine. Nitrosation occurs mainly by radical combination rather than using the potent nitrosating species nitrous anhydride (N2O3). Nitrosation predominates over nitration. Nitrosation is eliminated when [O2 ∙ − ] ≥ [ ∙ NO]. Nitration is relatively independent of excess ∙ NO vs. excess O2 ∙ − . Intermediate (not endproduct) nitrosothiol (GSNO) is formed when ∙ NO is in excess over O2 ∙ − . The extremely rapid reaction between ∙ NO and O2 ∙ − produces peroxynitrite (ONOO − ), a potent oxidizing agent, which also react very rapidly with either thiol or CO2. The reaction of either ∙ NO or O2 ∙ − with ONOO − forms the potent nitrosating species nitrous anhydride (N2O3) or nitrogen dioxide ( ∙ NO2, respectively. The products of reaction of CO2 with ONOO − and homolysis of peroxynitrous acid, ∙ NO2 and ∙ OH, rather than ONOO − or ONOOH. Both ∙ NO2 and ∙ OH can react with excess ∙ NO and O2 ∙ − . The intermediate reactive products of CO2 reaction, CO3 ∙ − and ∙ NO2, disappear rapidly. Nevertheless, competition of cellular targets (mainly thiol) for reactive intermediate oxidants (CO3 ∙ − and ∙ NO2) is much more effective than that for excess ∙ NO and O2 ∙ − .
Reaction | flux of ∙ NO w.r.t. that of O2 ∙ − | ||
---|---|---|---|
vO2 ∙ − < v ∙ NO | vO ![]() | vO2 ∙ − < v ∙ NO | |
Oxidation | 98.6% | 99.3% | 99.6% |
Nitration | 0.2% | 0.1% | 0.1% |
Nitrosation | 1.2% | 0.6% | 0.4% |
The terms “nitrosative stress” and “nitrative stress” are used to denote damage from reactive nitrogen species without refering specifically to nitrosation and nitration. The expression “nitroxidative stress” enables to distinguish an oxidative from a purely nitrosative or nitrative cause [1249]. Major agents are O2 ∙ − , 1O2, H2O2, and ∙ OH for oxidative stress and ONOO − , CO3 ∙ − , and ∙ NO2 for nitroxidative stress.
10.6.2.1 Reactants of Peroxynitrite
Peroxynitrite, a strong oxidant, directly reacts at a relatively slow rate with thiols,110 lipids, amino acids, DNA bases, and low-molecular-weight anti-oxidants [1168].
Proteins
Peroxynitrite interacts with proteins that contain transition metals. Peroxynitrite rapidly reacts with (inactivates) iron–sulfur domain-containing enzymes (e.g., mitochondrial aconitase Aco2) as well as zinc–sulfur motif-containing enzymes (e.g., NOS3 and alcohol dehydrogenase) [1168].
The direct reaction of peroxynitrite with transition metal centers pertains to the fastest reaction with peroxynitrite. Peroxynitrite thus modifies proteins that contain a heme prosthetic group, such as hemoglobin, myoglobin, and cytochrome-C by oxidizing ferrous heme into its ferric form.
Peroxynitrite can also inactivate NOS2 by oxidative modification of its heme group, thereby priming a negative feedback of peroxynitrite generation.
Amino Acids
Peroxynitrite may also change protein structure by reacting with amino acids, such as cysteine oxidation and tyrosine nitration [1168]. Peroxynitrite reacts directly with cysteine, methionine, and tryptophan residues. It also targets transition metal centers and selenium-containing amino acids.
In addition, free radicals arising from peroxynitrite homolysis111 such as hydroxyl, nitrogen dioxide, and carbonate radical (O=C(O ∙ )O − ) formed in the presence of carbon dioxide, also react with protein moieties.
Cysteine Oxidation
Peroxynitrite reacts with thiols, particularly with the anion form (R–S − ) to generate an intermediate sulfenic acid (R–SOH), which then reacts with another thiol, forming a disulfide (R–S–S–R).
Thiols may also be oxidized by radicals formed from peroxynitrite, thereby producing thiyl radicals (R–S ∙ ). Thiyl radicals may react with oxygen and promote oxidative stress. They also react with NO to form nitrosothiols, or thionitrites (R–S–NO).
Enzyme Inhibition
The oxidation of certain cysteine residues by peroxynitrite inactivates many enzymes involved in cellular energetic metabolism, such as glyceraldehyde-3-phosphate deshydrogenase, creatine kinase, and complex-I (NADH dehydrogenase), -II (succinate dehydrogenase), -III (cytochrome-C reductase), and -V (ATP synthase) of the mitochondrial respiratory chain [1168]. In addition, tyrosine nitration also inactivates these enzymes.
Cysteine oxidation by peroxynitrite also leads to the inactivation of several protein Tyr phosphatases. In addition to protein-bound thiols, peroxynitrite can directly oxidize low-molecular-weight thiols, especially reduced glutathione (GSH). The latter scavenges peroxynitrite.
Enzyme Activation
Cysteine oxidation by peroxynitrite can also activate certain types of enzymes such as matrix metallopeptidases. Peroxynitrite activates the proenzymes (proMMP) via thiol oxidation and S-glutathiolation of the auto-inhibitory domain.
Cysteine oxidation by peroxynitrite stimulates the SRC family kinase hematopoietic cell kinase (HCK) in erythrocytes.
Tyrosine Nitration
Protein tyrosine nitration is a covalent protein modification mediated by reactive nitrogen species such as peroxynitrite anion and nitrogen dioxide, i.e., introduction of a nitro group (-NO2) into a target molecule. Tyrosine nitration is a selective process limited to specific tyrosine residues on a relatively small number of proteins.112
A nitro group (-NO2) is added to Tyr residues to form nitrotyrosines (TyrNO 2). Tyrosine nitration influences protein structure and function. Tyrosine does not react directly with peroxynitrite. During tyrosine nitration, a hydrogen atom (TyrH is first abstracted from tyrosine to form a tyrosyl radical (Tyr ∙ ) that quickly combines with NO2 ∙ to produce 3-nitrotyrosine. A second combination with another tyrosyl radical forms dityrosine [1168].
Radicals involved in the reaction may come from peroxynitrite homolysis (OH ∙ and NO2 ∙ ). However, radicals can be CO3 − ∙ and NO2 ∙ radicals produced by the reaction between ONOO − and CO2.
Tyrosine nitration is enhanced in the presence of transition metals due to the formation of secondary radicals at the metal center plus NO2 ∙ . Metalloproteins, such as heme-containing proteins (e.g., prostacyclin synthase) or Cu,ZnSOD and MnSOD may catalyze peroxynitrite-mediated tyrosine nitration.
Another mechanism of tyrosine nitration relies on the generation of the NO2 ∙ radical by various heme-peroxidases (mainly myeloperoxidase and eosinophil peroxidase) in the presence of hydrogen peroxide [1168].
Tryptophan, Methionine, and Histidine Oxidation
Peroxynitrite can directly oxidize methionine, thus forming methionine sulfoxide (MetSO), and to a lesser extent, ethylene and dimethyldisulfide. Conversely, ubiquitous peptide methionine sulfoxide reductase carries out the enzymatic reduction of methionine sulfoxide to methionine, thereby repairing oxidative damage and restoring normal activity.
Methionine oxidation can participate in immunity, via inactivation of glutamine synthase and chaperonin GroEL in bacteria [1168].113 Methionine oxidation also inhibits serpin-A1.114
Peroxynitrite can also oxidize tryptophan to yield Nformylkynurenine,115 oxindole, hydropyrroloindole, and nitrotryptophan [1168].
Peroxynitrite modifies histidine to create a histidinyl radical, which inactivates (Cu,Zn)SOD type of superoxide dismutase [1168].
Lipids
Peroxynitrite can cause lipid peroxidation in cell membranes, liposomes, and lipoproteins, as it extracts a hydrogen atom from polyunsaturated fatty acids [1168]. Resulting products include lipid hydroperoxyradicals, conjugated dienes (C n H2n − 2), and aldehydes (R–CHO).
These radicals attack neighboring polyunsaturated fatty acids, thereby generating additional radicals that amplify free radical reactions and subsequent degradations.
Peroxynitrite acts as a potent oxidizing agent for low-density lipoproteins. Peroxynitrite-modified LDLs bind with high affinity to scavenger receptors, thereby leading to the accumulation of oxidized cholesteryl esters and formation of foam cells, an early event in atherogenesis.
Last, but not least, peroxynitrite interacts with membrane lipids and can generate various nitrated lipids that can serve as signaling mediators, as well as intermediate products, such as isoprostanes116 and 4-hydroxynonenal (4HNE [C9H16O2]),117 which can further trigger secondary oxidative insults [1168].
Nucleic Acids
Peroxynitrite can cause oxidative modifications in both nucleobases and carbohydrate–phosphate backbone, thereby damaging DNA [1168]. Among the 4 nucleobases, guanine is the most reactive with peroxynitrite. The major product of guanine oxidation is 8-oxoguanine, which further reacts with peroxynitrite to form cyanuric acid and oxazolone. Guanine oxidation by peroxynitrite provokes guanine fragmentation, thereby leading to carcinogenesis.
Peroxynitrite can nitrate guanine to form 8-nitroguanine. Resulting abasic sites can then be cleaved by endonucleases and generate DNA single-strand breaks [1168].
Peroxynitrite may also degrade the sugar phosphate backbone by removing a hydrogen atom from the deoxyribose moiety, hence opening the sugar ring and creating DNA strand breaks.
10.6.2.2 Peroxynitrite in Mitochondria
Peroxynitrite may enter into mitochondria or be produced in mitochondria. Mitochondria can actually synthesize both nitric oxide using Ca
-sensitive mitochondrial NOS (mtNOS) and superoxide upon partial reduction of oxygen within the mitochondrial matrix due to the natural leak of electron from the respiratory chain. In mitochondria, nitric oxide reversibly inhibits cytochrome-C oxidase (complex IV of the electron transport chain), as it competes with oxygen for the binding site, thereby regulating oxygen consumption [1168]. Moreover, peroxynitrite nitrates (inhibits) MnSOD, thus preventing the breakdown of locally produced superoxide and amplifying the formation of peroxynitrite.

Peroxynitrite also inhibits most components of the electron transport chain: NADH dehydrogenase (complex I), succinate dehydrogenase (complex II), cytochrome-C reductase (complex III), and ATP synthase (complex V) using cysteine oxidation, tyrosine nitration, and damage of iron–sulfur centers [1168].
Furthermore, peroxynitrite impedes the activity of aconitase of the tricarboxylic acid cycle in the mitochondrial matrix as well as mitochondrial creatine kinase in the intermembrane space.
Peroxynitrite targets nicotinamide nucleotide transhydrogenase that generates NADPH from NADH and NADP + , thereby reducing the mitochondrial ability to regenerate the reduced form of glutathione (GSH) [1168].
10.6.2.3 Peroxynitrite and Cell Signaling
Peroxynitrite nitrates tyrosine residues and can impair TyrP-dependent signaling. Phosphorylation of critical Tyr residues can be prevented by peroxynitrite-mediated Tyr nitration, thereby blocking signaling [1168].
On the other hand, TyrP-dependent signaling can be activated by peroxynitrite [1168]. In particular, peroxynitrite stimulates MAPK superfamily members (Table 10.24). Phosphotyrosine signaling stimulation results either from the inhibition of protein TyrP phosphatases (PTP) or activation of protein Tyr kinases (PTK).
Table 10.24.
Main signaling pathways influenced by peroxynitrite in certain explored cell types (Source: [1168]; EGFR: epidermal growth factor receptor protein Tyr kinase; ERK: extracellular-regulated kinase; JNK: Janus kinase; MAPK: mitogen-activated protein kinase; NFκB: nuclear factor-κB; NTRK: neurotrophic protein Tyr receptor kinase; PDGFR: platelet-derived growth factor receptor protein Tyr kinase; PI3K: phosphatidylinositol 3-kinase; PKB(C): protein kinase-B(C); TNFRSF: tumor-necrosis factor receptor superfamily member).
Mediators | Effect of | Explored cell types |
---|---|---|
peroxynitrite | ||
EGFR | Activation | Fibroblast, myofibroblast |
Inhibition | Colonic (Caco2B) cell | |
PDGFR | Activation | Fibroblast |
NTRKb | Activation | Fibroblast |
TNFRSF6a | Inhibition | Hepatocyte |
Src | Activation | Erythrocyte, endothelial cell, astrocyte |
ERK | Activation | Cardiomyocyte, neuron, fibroblast, neutrophil, |
vascular smooth muscle cell, endothelial cell | ||
JNK | Activation | Alveolar and bronchial cells, endothelial cell, |
cardiomyocyte, fibroblast | ||
P38MAPK | Activation | Neurons, endothelial and bronchial cells, |
vascular smooth muscle cell, cardiomyocyte, | ||
hepatocyte | ||
PKC | Activation | Cardiomyocyte, endothelial cell |
Inhibition | Neuron | |
PI3K–PKB | Activation | Endothelial cells, hepatocyte, fibroblast |
Inhibition | Endothelial cell, macrophage, adipocyte, | |
retinal cell | ||
NFκB | Activation | Neutrophil, monocyte |
Inhibition | Cardiomyocyte |
Plasmalemmal and Cytosolic PTKs
Peroxynitrite anion (ONOO − ) is structurally similar to phosphate anion (PO4 3 − ). Therefore, protein Tyr phosphatases are vulnerable to peroxynitrite. On the other hand, protein Tyr kinases can be directly activated by peroxynitrite. Receptor protein Tyr kinases, particularly growth factor receptors EGFR and PDGFR, undergo Tyr phosphorylation upon exposure to oxidants. However, peroxynitrite-mediated nitration of EGFR can prevent its phosphorylation in a certain type (Caco2) intestinal cells [1168].
Cytosolic protein Tyr kinases are also targeted by peroxynitrite. In particular, SRC family kinases can be activated by peroxynitrite at least in erythrocytes and endothelial cells. In erythrocytes, hematopoietic cell kinase (HCK) is activated by peroxynitrite via cysteine oxidation [1168]. Another SRC family kinase, Lyn kinase, is activated via the inhibition of the binding of auto-inhibitory Tyr527 C-terminal domain to the interaction SH2 domain.
MAPK Module
Components of the MAPK module (ERKs, JNKs, and P38MAPKs) can be activated by oxidants and free radicals. Peroxynitrite can potently stimulate ERK in endothelial and vascular smooth muscle cells, fibroblasts, cardiomyocytes, neutrophils, and neurons, using cell-specific mechanisms [1168]. Activation of JNK kinases by peroxynitrite has been observed in endothelial cells subjected to flow. Peroxynitrite is very efficient in activating P38MAPK in cardiomyocytes, endothelial and vascular smooth muscle cells, bronchial epithelial cells, and neurons [1168]. In particular, peroxynitrite stimulates P38MAPK: (1) via MAP3K3 and MAP3K6 following ERK-dependent activation of cytosolic phosholipase-A2 in human bronchial epithelial cells; (2) via activated Ca
–calmodulin kinase CamK2 and Src in PC12 cells; and (3) via the release of Zn
in neurons.


The PI3K–PKB Axis
In human skin fibroblasts, peroxynitrite can support PDGFR–PI3K–PKB signaling. On the other hand, peroxynitrite can preclude the PI3K signaling and PKB activation in several cell types, in particular endothelial cells. The chemical microenvironment may affect the response to peroxynitrite [1168].
PKC Isoforms
In cardiomyocytes, peroxynitrite can activate PKCε during ischemic preconditioning. In endothelial cells, stimulation of PKC by peroxynitrite is associated with the activation of cytosolic phospholipase-A2 and an enhanced release of vasoactive mediators. On the other hand, peroxynitrite reduces the activity of PKCα, PKCβ, PKCε, and PKCζ in neurons [1168].
NFκB
Nuclear factor-κB is a transcriptional activator of inflammation and anti-apoptotic genes. In addition to reactive oxygen species, peroxynitrite can activate or repress NFκB [1168].
10.7 Hippo Signaling (STK3–STK4 Pathway)
The Hippo signaling pathway participates in the control of organ size, during the body’s development as well as tissue regeneration, as it restricts cell proliferation and promotes cell elimination.118 The appropriate number of cells, hence size of the body’s tissues and organs, is actually determined by coordinated cell growth, proliferation, and apoptosis. Disruption of these processes can cause cancers.
The Hippo kinase is the Drosophila homolog of mammalian protein Ser/Thr kinases STK3 and STK4119 (Sect. 5.1) that controls both cell proliferation and death. Kinases STK3 and STK4 are named tumor suppressors, as they promote apoptosis in particular in hepatocytes [1250].
Another Hippo signaling component, the Salvador ortholog Sav1 (or WW45) facilitates activation of STK3 and STK4 kinases. In mammals, each component of the Hippo cascade regulates cytosolic or nuclear location of mammalian yorkie orthologs Yes-associated protein YAP1 and WW domain-containing transcription regulator WWTR1 (or transcriptional coactivator with PDZ-binding motif [TAZ]) to control cell proliferation and survival, as well as self-renewal and differentiation of stem and progenitor cells. In addition, the Hippo pathway crosstalks with other signaling axes, such as those of Notch, sonic Hedgehog, and Wnt morphogens.
10.7.1 Hippo Signaling Components
Known components of the Hippo (STK3–STK4) pathway include (Fig. 10.3 and Tables 10.25 to 10.27): (1) receptor Fat1 protocadherin and the other non-classical cadherin Dachsous from adjacent cells that can modulate the Hippo axis;120 (2) adaptors neurofibromatosis NF2 (mammalian homolog of Merlin) and FERM domain-containing protein-6 (FRMD6), or Expanded-1 (Ex1; human ortholog of Expanded) that operates in parallel with NF2; (3) scaffold protein WWC1 (or KiBra) that tethers to both FRMD6 and NF2 to form an apical scaffold that promotes Hippo axis activity; (4) kinases STK3 and STK4 (human ortholog of Hippo); (5) scaffold protein WW45 (or Salvador-1 [Sav1]; ortholog of Drosophila Salvador) that binds to kinases STK3/4 and LaTS (active STK3/4–WW45 platform); (6) large tumor suppressor kinases LaTS1 and LaTS2 (mammalian homologs of Warts) that are phosphorylated (activated) by STK3 and STK4 complexed with WW45; (7) transcriptional coactivator YAP1 (human ortholog of Yorkie), which is phosphorylated (stimulated) by LaTS kinases complexed with MOB1, to activate genes responsible for cell proliferation and survival, as well as its paralog WWTR1; and (8) other components, such as Ras and Ras association domain-containing family proteins (RASSF) and microRNA Bantam. In addition to this canonical pathway (PCdh–NF2–STK3/4–LaTS1/2–YAP1/WWTR1), Hippo signaling relies on crosstalks with other signaling cascades.

Fig. 10.3
Signaling by Hippo regulator (STK3–STK4 pathway; Source: [1251]). The Hippo cascade controls tissue growth, as it impedes cell proliferation and promotes apoptosis. The Hippo signaling requires 2 related cortical band-4.1 proteins, the tumor suppressor neurofibromatosis NF2 (Merlin homolog), a membrane-cytoskeleton scaffold, and FERM domain-containing protein-6 (FRMD6 or Expanded-1 [Ex1]; human ortholog of Expanded), the upstream effectors of the STK3/4 pathway targeted by protocadherin Fat1. Activity of NF2 depends on its phosphorylation by P21-activated kinase and activation by Rac GTPase. Expanded-1 homolog is a potent inhibitor of Yes-associated protein (YAP1) in parallel to YAP1 phosphorylation and cytosolic sequestration by 14-3-3 protein. Proteins NF2 and Expanded-1 promote the phosphorylation (activation) of large tumor suppressor kinase (LaTS) by activated protein Ser/Thr kinases STK3 and STK4 (a.k.a. mammalian sterile-20-like kinases MST2/1, i.e., mammalian homolog of Hippo). The scaffold protein WW domain-containing protein WW45 (mammalian homolog of Salvador) binds to LaTS and STK3/4 to favor LaTS phosphorylation. Activation of LaTS also requires plasmalemmal localization of Mps one-binder enzyme (MOB) as tumor suppressor (MATS1 or MOB kinase activator-like protein MOBKL1b). Once activated by STK3 and/or STK4 kinases, LaTS phosphorylates (inactivates) YAP1 transcriptional coactivator. The latter regulates the activity of various transcriptional regulators, such as P73 and P53-binding protein-2. Protocadherin Fat1 acts as a transmembrane receptor for protocadherin Dachsous to trigger the Hippo pathway.
Table 10.25.
Components of the Hippo (STK3–STK4) pathway. (Part 1) Cell-surface mediators (protocadherins). Some mediators of the Hippo pathway in Drosophila melanogaster, in which it was originally discovered, can have several homologs in mammalian cells. These paralogs sometimes play redundant roles, but in most cases have distinct properties.. The Hippo pathway regulates organ size. It is composed of: (1) plasmalemmal regulators, such as cell adhesion molecules and cell polarity complexes; (2) a cytoplasmic cascade with 2 protein Ser/Thr kinases and their regulators and adaptors; and (3) a transcription coactivator (CDHF: cadherin family member; CDHF7 is also called cadherin ME5 and cadherin-related homolog CdhR8; MGEF1: multiple EGF-like domain-containing protein; PCdh: protocadherin).
Mammals | Drosophila | |
---|---|---|
Type | Other aliases | |
Dchs1 | Cdh19 (25), CdhR6, PCdh16 | Dachsous |
Dchs2 | Cdh27, CdhR7, PCdh23, PCdhJ | Dachsous |
Fat1 | CDHF7, CdhR8, ME5 | Fat |
Fat2 | CDHF8, CdhR9, MEGF1 | Fat |
Fat3 | CDHF15, CdhR10, | Fat |
Fat4 | CDHF14, CdhR11, FatJ | Fat |
Table 10.26.
Components of the Hippo (STK3–STK4) pathway. (Part 2) Cytoplasmic mediators (CDHF: cadherin family member, CDHF7 is also called cadherin ME5 and cadherin-related homolog CdhR8; DTEF: divergent TEF; ETFR: enhancer of transcriptional factor-related; FRMD: FERM domain-containing protein, or Expanded-1 [Ex1]; KiBra: kidney and brain protein; KPM: kinase phosphorylated during mitosis; LaTS: large tumor suppressor kinase; MATS: Mps one-binder (MOB) as tumor suppressor; MATS1 is also named MOB1a and MOB kinase activator-like protein MOBK1Lb; MST: mammalian Ste20-like kinase; NF2: neurofibromatosis-2; RTEF: related to TEF; STK: protein Ser/Thr kinase; TAZ: transcriptional coactivator with PDZ-binding motif; TCF: transcription factor; TCF13L: transcription factor-13-like; TEAD: TEA domain-containing family member; TEF: transcriptional enhancer factor; VgL: Vestigial-like protein; ViTo: vestigial- and Tondu-related protein; WW45: 45-kDa WW domain-containing protein; WWC: WW and C2 domain-containing protein; WWTR: WW domain-containing transcription regulator; YAP: Yes Tyr kinase-associated protein).
Mammals | Drosophila | |
---|---|---|
Type | Other aliases | |
Kinases | ||
LaTS1 | Warts (Wts) | |
LaTS2 | KPM | Warts |
STK4 | MST1 | Hippo |
STK3 | MST2 | Hippo |
Adaptors, scaffolds, and activators | ||
FRMD6 | Ex1 | Expanded |
MATS1 | MOB1a (4b), MOBK1Lb | MATS |
MATS2 | MOB1b (4a), MOBK1La | MATS |
NF2 | Merlin | |
WW45 | WWP4, Sav1 | Salvador |
WWC1 | KiBra | |
Transcriptional coactivators | ||
TEAD1 | TEF1, TCF13 | Scalloped |
TEAD2 | TEF4 | Scalloped |
TEAD3 | TEAD5, TEF5, DTEF1, ETFR1 | Scalloped |
TEAD4 | TEF3, EFTR2, RTEF1b, TCF13L1 | Scalloped |
VgL1 | Tondu (Tdu) | Vestigial |
VgL2 | ViTo1 | Vestigial |
VgL3 | Vestigial | |
WWTR1 | TAZ | Yorkie (Yki) |
Taffazin | ||
(Saccharomyces cerevisiae) | ||
YAP1 | YAP2, YAP65 | Yorkie |
Table 10.27.
Effector complexes of Hippo signaling. Upon stimulation, several molecular coupling platforms are built along the Hippo axis in different cellular compartment in the canonical pathway. In addition, the Hippo pathway crosstalks with other signaling axes, especially those involved in morphogenesis (TGF, Notch, Hedgehog, and Wnt).
Site | Effectors |
---|---|
Cell surface and cortex | Fat–Dachsous–NF2 |
Cytoplasm | STK3–STK4–WW45–LaTS1/2 |
LaTS1–LaTS2–MOB1–YAP1/WWTR1 | |
Nucleus | YAP1–VgL–TEAD |
WWTR1–TEAD |
Cell proliferation during embryogenesis and childhood becomes restricted in adulthood. Cell division then serve for tissue maintenance. Kinases STK3 and STK4 particularly promote apoptosis when they are primed by irradiation and activated by P53 [1251]. Activation of P53 by these kinases is modulated by proteins of the Ras and Ras association domain-containing family (RASSF).121 Hippo signaling restrains cell proliferation, as it prevents activation of YAP1 transcriptional coactivator.
The Hippo pathway comprises Fat1 cadherin and microRNA Bantam. Protocadherin Fat1 stabilizes Large tumor suppressor kinases (LaTS).122
The Hippo signaling may repress microRNA Bantam that elicits tissue growth and patterning and inhibits apoptosis. On the other hand, miR372 and miR373 target transcripts of large tumor suppressor kinases. The latter then cannot phosphorylate (inactivate) the 2 transcription coactivators YAP1 and WWTR1 [1253].
10.7.1.1 Signaling Crosstalk
Signaling efficiency in cells compels many proteins to function in various modes and possible locations. Proteins WWTR1 and YAP1 acts with other mediators to regulate transcription in response to activation of the Hippo pathway. Factors WWTR1 and YAP1 can move between the nucleus and cytoplasm.
Moreover, WWTR1 participates in the Wnt pathway that controls cell fate in developing and remodeling tissues. It interacts directly with Disheveled. It impedes the phosphorylation of Disheveled by casein kinases CK1δ and CK1ε. Consequently, it restricts the canonical Wnt–β-catenin pathway [1254].
Hippo signaling restrains cardiomyocyte proliferation, thereby controlling the heart size in developing mice. In cardiomyocytes, Hippo regulates the genes that encode transcription factors Sox2, Snai2, and lung-derived MycL1 (and Myc in hepatocytes) as well as those that encode the cell cycle regulators cyclin-D1 and CDC20 [1256]. Effector YAP1 interacts with β-catenin on Sox2 and Snai2 genes [1256]. However, phosphorylation of YAP1 triggered by Hippo signaling provokes YAP1 sequestration into the cytoplasm. Activity of Hippo- and Wnt-regulated genes is thus repressed.
Agent WWTR1 is also implicated in signaling by transforming growth factor-β [1255]. In human embryonic stem cells, WWTR1 interacts with SMAD complexes. In particular, YAP1 binds SMAD1 and supports subsequent gene transcription.
Furthermore, WWTR1 is a component of the ubiquitin ligase complex SCFβTRCP that degrades calcium-permeable cation channel polycystin-2 [1257].
In Drosophila, Hippo signaling can activate Notch signaling. Sonic Hedgehog increases YAP1 expression and promotes its nuclear localization; subsequently, YAP1 primes expression of Gli2 transcription factor [1258].
Moreover, YAP1 targets the amphiregulin gene; this ligand of epidermal growth factor receptor activates EGFR signaling [1259].
In Drosophila melanogaster, the growth suppressor Yorkie, a transcriptional regulator, binds to the MYC gene [1260]. Conversely, transcription factor MyC can regulate Yorkie expression, i.e., its own cellular level. Growth-promoting MyC represses Yorkie expression using both transcriptional and post-transcriptional mechanisms.
10.7.1.2 Intercellular Contacts – Protocadherins
The Hippo pathway is activated by intercellular contacts and engagement of protocadherin Fat1, the largest cadherin.123 Protocadherin Fat1 acts as a receptor to regulate cell growth and polarity [1262]. Protocadherin Dachsous can extracellularly interact with Fat1 protocadherin.
Protocadherins Fat1 and Dachsous indeed form heterodimeric bridges between adjacent cells.124 Kinases STK3 and STK4 transfer signals from these atypical cadherins of the Fat and Dachsous categories at the plasma membrane to intracellular kinase LaTS1 to regulate transcriptional coactivators.
10.7.1.3 STK3 and STK4 Kinases
Substrates of STK3 (Sect. 5.1.4.1) and STK4 (Sect. 5.1.4.2) kinases include not only components of the Hippo pathway, such as LaTS kinases, WW45, and Mps one binder kinase (MOB) activator-like proteins, but also STK38, NeK2 (never in mitosis gene-A [NIMA]-related kinase-2), and JNK (activation) kinases, histone-2AX and -2B, and FoxO factor [1258] (Table 10.28).
Table 10.28.
Mediators of the Hippo pathway and their partners and substrates. (Source: [1043, 1258]; HTRa2: mitochondrial high temperature requirement serine peptidase-A2; NeK: never in mitosis gene-A (NIMA)-related kinase; RCC: regulator of chromosome condensation). Mediators of the Hippo pathway interact with various proteins, such as Na + –H + exchanger regulatory factor NHERF1, or SLC9a3R1, 14-3-3 protein, HER4 receptor, as well as P73, Runx1, Runx2, SMAD7, and TEAD1 factors.
Components | Interactors |
---|---|
STK3/4 | LaTS, WW45, MOBKL1a/1b, YAP1, WWTR1 |
NeK2, JNK, RASSF6, RCC1, FoxO, histone-2AX/2B | |
STK3 | histone-1H4A–histone-1H4E |
STK4 | PKB1, Aurora-B, STK38/38L, RASSF5 |
LaTS1 | CDK1, LIMK1, STK3/4, HTRA2, zyxin |
LaTS2 | STK3/4, YAP1, MOBKL1a, PP1r13b, ajuba (Jub) |
YAP1 | Abl, PKB, RASSF1a, 14-3-3, SLC9a3R1, |
cyclin-E (in Drosophila), cyclin-D1 in vertebrates), | |
P73, PML, Runx, TEAD | |
WWTR1 | LaTS2, GSK3β, TEADs |
WWC1 | NF2, FRMD6 |
NF2 | PAK, PP1r12, CRL4–DCAF1 ligase |
Kinases STK3 is cleaved by caspase-3 to then undergo an irreversible autophosphorylation. Its full activation indeed requires both caspase-mediated cleavage and phosphorylation. Kinase STK4 mediates apoptosis upon cleavage, autophosphorylation and nuclear translocation [1264].
Kinase PKB phosphorylates STK3 (Thr117 and Thr384) and STK4 (Thr120 and Thr387), hence impeding their cleavage. Kinase STK4 is dephosphorylated by PHLPP1 and PHLPP2 phosphatases [1258]. Agent RASSF1 promotes STK3 and STK4 autophosphorylation.
Activity of STK3 and STK4 kinases is controlled by cRaf and phosphatases. Protein phosphatase-2 activates STK4 kinase. Connection between STK3 and cRaf is promoted by PKB [1258]. On the other hand, RASSF1a (RASSF1a and RASSF1c being major RASSF1 isoforms) relieves STK3 inhibition by cRaf kinase. In addition, STK3 and STK4 kinases and RASSF6 mutually inhibit each other. Kinase JNK, a substrate of STK4, phosphorylates STK4 (Ser82) to enhance its activity [1258].
Kinase STK4 phosphorylates (inactivates) Aurora-B as well as P53 transcription factor; it also inhibits PKB1 enzyme.
Members of the RASSF family (RASSF1–RASSF10), are components of the Hippo pathway.125 Agent RASSF1a complexes with STK3, WW45, and LaTS1 components [1258].126
The other family member RASSF6 interacts with STK3 and STK4, but it can support apoptosis independently of the Hippo pathway. Both RASSF1a and RASSF6 participate in apoptosis partly via modulator of apoptosis protein MOAP1. Kinase STK3 precludes RASSF6–MOAP1 interaction, thereby hindering RASSF6-mediated apoptosis. Conversely, RASSF6 suppresses STK3 activity, possibly by inhibiting homo-oligomerization [1258].
10.7.1.4 LaTS Kinases
Mammalian LaTS kinases pertain to the nuclear DBF2-related (NDR) family. Member NDR1, or STK38, is involved in the regulation of centrosome and chromosome alignment. Both NDR1 and NDR2 (STK38L) are activated by STK4 to mediate apoptosis downstream from the RASSF1a–STK4 module [1258].
Both LaTS1 and LaTS2 localize to the centrosome. The centrosomal location of LaTS2 is controlled by Aurora-A [1258]. During mitosis, LaTS1 interacts with zyxin, a zinc-binding phosphoprotein that accumulates in focal adhesions and along the actin cytoskeleton, to ensure a normal mitosis progression.
Kinase LaTS2 associates with ajuba, a regulator of cell proliferation and differentiation, and recruits γ-tubulin to centrosomes during mitosis. It also binds to DM2 and inhibits its ubiquitin ligase activity, thereby stabilizing P53 factor. Enzyme LaTS2 phosphorylates inhibitory subunit of protein phosphatase-1 (PP1r13b) to induce its nuclear translocation. In the nucleus, P53 then targets promoters of cell cycle regulator genes rather than those of pro-apoptotic genes [1258]. On the other hand, cytoplasmic PP1r13b prevents the phosphorylation by LaTS1 of YAP1 and WWTR1 and increases their nuclear levels to promote cell survival.
10.7.1.5 YAP1 and WWTR1 Transcriptional Coregulators
Transcriptional coregulators YAP1 and WWTR1 share interactors. Yes-associated protein-1 binds to the Src homology SH3 domain of Yes kinase proto-oncogene product. Molecule YAP1 is characterized by domains with 2 tryptophans (WW) that enables binding of proteins with a PPXY sequence, among other between-protein interaction domains; YAP1 is a founding member of the family of WW domain-containing proteins. Protein YAP1 links to members of the P53 family (P73α–P73β and P63), SMAD7, and Runx2 factors, as well as HER4 receptor [1267]. Proteins that interact with YAP1 N-terminus include heterogeneous nuclear ribonucleoprotein hnRNPu, TEA domain-containing transcription factors, and LaTS kinases. Moreover, YAP1 C-terminus contains a PDZ-binding motif for binding to PDZ domain-containing proteins.
Transcriptional coactivator YAP1 is the nuclear effector of STK3 and STK4 that targets cyclin-E (in Drosophila), among other mediators [1268]. Transcriptional coactivator YAP1 promotes epithelial–mesenchymal transition, hence malignant transformation. Regulator YAP also operates as a secondary coactivator of steroid hormone receptors to enhance their transcriptional activity.
Phosphorylation of YAP1 by Abl kinase switches YAP1 activity from a proliferative to an apoptotic mode [1269]. Upon DNA damage, phosphorylated YAP ceases its interaction with Runx transcriptional factor, thus precluding the expression of Itch Ub ligase to favor P73 accumulation. Furthermore, its interaction with P73 via RASSF1a initiates expression of pro-apoptotic genes. Mediator YAP contributes to 2 distinct tumor suppressor pathways: via promyelocytic leukemia (PML)128 and P73 transcription factors.
YAP1 Subcellular Localization
YAP1 Cytoplasmic Sequestration
In some cell types, YAP1 localizes mainly to the cytosol, as it is phosphorylated by PKB kinase. Once YAP1 is phosphorylated by PKB, YAP binds to 14-3-3 protein, thereby being sequestered in the cytoplasm. Kinase LaTS phosphorylates YAP1, thus labelling it for degradation. The Crumbs homolog complex129 (MPP5–InaDL–Lin7c–Angiomotin) assembled at tight junctions interacts with YAP1 and also sequesters it in the cytosol. In addition, YAP1 connects to SLC9a3R1 regulator and can then be anchored to the plasma membrane. Tight junction proteins ZO1 and ZO2 bind WWTR1; the latter, which can lodge in the nucleus, also tethers to YAP1 factor.
When LaTS kinases phosphorylate YAP1, the latter is further phosphorylated by casein kinases CK1δ and CK1εfor ubiquitination and subsequent degradation.
YAP1 Nuclear Accumulation
In other cell types and context, YAP1 nuclear accumulation promotes P73-dependent transcription of pro-apoptotic genes. Kinase Abl phosphorylates YAP1, thereby supporting the association of YAP1 with P73 factor [1258]. Factor YAP1 competes with ubiquitin ligase Itch for P73 binding. Promyelocytic leukemia transcription factor (PML) recruits YAP1 and stabilizes P73 factor via sumoylation. As PML is a target of P73, a positive feedback loop exists between these 2 molecules.
Role of YAP1 and WWTR1 in Mechanotransduction
Activity of YAP1 and WWTR1 on cognate genes, such as those that encode connective tissue growth factor (CTGF or CCN2) and cardiac ankyrin repeat domain-containing protein AnkRD1, depends on the rheology of the matrix. Expression of the Ctgf and ANKRD1 genes is observed in human cells cultured on a stiff material, but not on soft matrices [1270]. In the latter case, YAP1 and WWTR1 lodge predominantly in the cytoplasm. Tension of the actin cytoskeleton transmitted by stress fibers enables localization and retention of YAP1 and WWTR1 in the nucleus. This action of YAP1 and WWTR1 is mediated by Rho GTPase that acts on the actomyosin cytoskeleton, but not by the NF2–STK3/4–LaTS axis [1270]. Therefore, YAP1 and WWTR1 operate as mediators of mechanical signals exerted by the cell environment.
10.7.1.6 Scaffold WW45 – Salvador-1
Scaffold protein WW45 (or Salvador-1 homolog) binds to STK3, STK4, and LaTS1 kinases. These kinases synergistically phosphorylate YAP1 and WWTR1, respectively, hence causing their cytoplasmic sequestration to suppress tissue growth.130 Both YAP1 and WWTR1 regulate gene expression via their cognate transcription factor TEA domain transcription factors TEAD1, also called transcription enhancer factor TEF1 (homolog of Drosophila Scalloped) [1269].131
Activity of WWTR1 is not only regulated by LaTS2 kinase, but also by glycogen synthase kinase GSK3β that primes its ubiquitination for proteolysis [1269]. Yes-associated protein forms a transactivation complex with TEF1 and its cofactors Vestigial-like proteins (VgL1–VgL3; homologs of Drosophila Vestigial).132 Vestigial-like proteins can induce the expression of transcription factor E2F1 [1273].
10.7.1.7 WWC1
Human WW domain-containing protein WWC1 modulates the activation of Hippo signaling. This early component of the Hippo pathway links to Hippo-related, cortical proteins FRMD6 and NF2 and promotes FRMD6–NF2 binding [1274–1276]. The FRMD6–NF2–WWC1 complex localizes to the apical region of epithelial cells to regulate the Hippo cascade. In addition, in cell cultures, WWC1 complexes with LaTS1 and causes a marked reduction in YAP1 phosphorylation without affecting the LaTS–YAP1 interaction [1275]. Protein WWC1 can also stimulate the phosphorylation of LaTS1 and LaTS2 enzymes.
10.8 Coregulators
Signaling networks create transient and focal points of enzyme activity that disseminate in a spatially and temporally controlled manner within the cell signals brought by neurotransmitters, hormones, and growth factors. Compartmentation is dictated by certain mediators, themself supervised by intracellular messengers.
Manifold cell functions can be specifically and efficiently controlled by a limited number of proteins over various distinct enzymes. Cell activities, particularly effector responses to receptor stimulations, are processed by between-protein interactions required in signaling molecules. Regulators and targets must be appropriately located to achieve the cell functions, especially in the heart for the spatiotemporal regulation of firing action potentials by nodal cells or secretion of hormones by cardiomyocytes.
Signaling networks are particularly associated with cytosolic foci of enzyme activity that transmit the action of messengers in the cytosol. Anchoring proteins (or anchor proteins) yield a framework that orients these enzymes toward selected substrates to elicit a specific response.
Coregulators participate in signaling by organizing molecular interactions and determining pathway dynamics.
A-kinase-anchoring proteins (AKAP) control the location and substrate specificity of protein kinase-A. Protein kinase-A1 resides mainly free in the cytoplasm, where it has a high affinity for cAMP, whereas protein kinase-A2 localizes more precisely, as it couples to A-kinase-anchoring proteins. The latter are scaffold proteins that determine the spatial organization of signaling pathways, as it associates PKA with its substrates. A-kinase-anchoring proteins possess various binding domains that enable the identification and anchoring of specific targets in given subcellular regions. On the other hand, inhibition of PKA–AKAP interactions modulates the PKA signaling.
Annexins have many partners implicated in signaling cascades and operate in interactions between the cell membrane and cytoskeleton.
Like AKAPs that connect to protein kinase-A (Sect. 10.8.4), a G-kinase-anchoring protein (GKAP1) tethers to protein kinase-G. Alias GKAP1 avoid confusion with guanylate kinase-associated protein (GKAP) implicated in the formation and maintenance of molecular platforms in synapses of the nervous system.
Specific anchoring proteins exist also for protein kinase-C, receptors for activated protein kinase-C (RACK; Table 10.29).
Table 10.29.
Specific anchoring proteins for protein kinase-A, -C, and -G. These anchoring proteins are aimed at ensuring the accessibility and phosphorylation of specific substrates at given subcellular compartments.
Type of protein kinase | Specific anchoring proteins |
---|---|
Protein kinase-A | A-kinase-anchoring proteins |
(AKAP1–AKAP14) | |
Protein kinase-C | Receptors for activated protein kinase-C |
(RACK1–RACK2) | |
Protein kinase-G | G-kinase-anchoring protein |
(GKAP1) |
10.8.1 Receptors for Activated C-Kinase
Isozymes of the PKC family (Sect. 5.2.7) are split into 3 subfamilies: (1) the subfamily of conventional enzymes that includes PKCα, PKCβ1, PKCβ2, and PKCγ, which require diacylglycerol, calcium, and phospholipid for activation;133 (2) the subfamily of novel enzymes that encompasses PKCδ, PKCε, PKCη, and PKCθ, which necessitate diacylglycerol for activation; and (3) the subfamily of atypical enzymes that comprises PKCζ, PKCι, PKN1, and PKN2, which involve neither diacylglycerol nor calcium for activation.
Different receptor for activated C-kinases target different PKC isoforms. The RACK proteins bind activated PKC in a selective manner, but are not substrates of PKC isozymes. Among the RACK family members, RACK1 is selective for activated PKCβ2, RACK2 for PKCε [1277]. The multifunctional chaperone glycoprotein C1QBP (complement component-1, Q-subcomponent-binding protein)134 is a PKC-binding protein that interacts with PKCα, PKCδ, PKCζ, and protein kinase-D1 (PKD1 or PKCμ).
Receptors for activated C-kinase support the translocation of each PKC isoform to a specific subcellular compartment. For example, RACK activates mitochondrial PKCε that interacts directly with mitochondrialATP-sensitive K + channel at the inner mitochondria membrane [1278]. The activity of this functional signaling module is impeded by protein phosphatase-2. Receptors for activated C-kinase also enhance the duration of PKC activation, thereby increasing substrate phosphorylation.
On the other hand, pseudo-rack regions on PKC, which are homologous to RACK protein, interacts with the RACK-binding site and stabilizes the enzyme in its inactive state. Activators that match this sequence interfere with the intramolecular interaction and, thus, activate PKC enzyme.
Receptors for activated protein kinase-C constitute a family (Table 10.30). Receptor for activated C-kinase RACK1 is homologous to the Gβ subunit guanine nucleotide-binding (G) proteins. The RACK proteins serve as adaptors not only for PKCβ1 and PKCε, but also for several other signaling mediators. In particular, RACK1 also binds to integrin-β1, dynamin-1, RasA1 GAP, Src and Fyn kinases, PDE4d5 phosphodiesterase, and phospholipase-Cγ [1279], as well as PTPRm phosphatases, cyclin-A1, STAT1 and NR3c4 transcription factors, and AT1, CSF2Rβ, and IfnαR2 receptors[251]. In addition, RACK2 is a coated vesicle component involved in vesicular transfer.
Table 10.30.
Family of receptors for activated protein kinase-C (bHLHb(e): class-B(E) basic helix–loop–helix protein; CoP: coatomer protein; GNβ2L1: guanine nucleotide-binding (G) protein-β polypeptide-2-like-1; Olig2: oligodendrocyte lineage transcription factor-2; PrKCBP: protein kinase C-binding protein ZMYND8: zinc finger, MYND-type domain-containing protein-8).
Type | Other aliases |
---|---|
RACK1 | GNβ2L1 |
RACK2 | β′-CoP, RACKε |
RACK7 | PrKCBP1, ZMYND8 |
RACK17 | PrKCBP2, Olig2, bHLHb1, bHLHe19 |
10.8.2 G-Kinase-Anchoring Protein
Second messenger cGMP is produced in response to nitric oxide and natriuretic peptides. The NO–cGMP and NP–cGMP pathways intervene in numerous processes in the nervous, cardiovascular, and immune systems. Agent cGMP is involved in the regulation of smooth muscle relaxation, platelet aggregation, intestinal secretion, and endochondral ossification via activated cGMP-dependent protein kinases-G (Sect. 5.2.9). The latter possesses 2 subtypes — PKG1 and PKG2 — that are encoded by distinct genes. In addition, PKG1 has 2 isoforms (PKG1α and PKG1β) that are generated by alternative splicing.
The cGMP-dependent signal transduction involves not only protein kinase-G, but also its substrates and regulators. Among regulators, G-kinase-anchoring protein-1 (GKAP1)135 interacts with PKG1α, but neither with PKG1β nor with PKG2 isozyme [1280].136
G-kinase-anchoring protein GKAP1 is specifically expressed in testis, more precisely spermatocytes and early round spermatids [1280]. Inside the cell, GKAP1 localizes to the Golgi body. In fact, PKG1α directly interacts with and phosphorylates GKAP1 to facilitate the translocation of PKG1α to the Golgi body in response to intracellular cGMP accumulation that causes a conformational change and releases PKG1α from GKAP1.137
10.8.3 Scaffolds of Synapses of the Central Nervous System
The GKAP–SAPAP/DLGAP (guanylate kinase-associated protein–SAP90/PSD95-associated protein/Disc large homolog-associated protein) family comprises scaffold proteins for assembling and maintenance of proteic signaling platforms associated with synaptic membranes.138
Members of the membrane-associated guanylate kinase (MAGUK) superfamily are organizers of cellular junctions.139 In particular, these molecular scaffolds structure ion channels and signaling molecules at the synaptic junction, especially the assembly and function of signaling complex at postsynaptic densities of excitatory synapses.
Among the MAGUK superfamily members, members of the PSD95 family, i.e., scaffold Disc large homologs (DLg1–DLg4; Table 10.31) anchor glutamate receptors to neuron terminals. NMDA (Nmethyl Daspartate)-type glutamate receptors interact directly with DLg4 that organizes a cytoskeletal–signaling complex at the postsynaptic membrane. Disc large homolog-4 interacts not only with GluN2a and GluN2b, but alsoKV1 channels, and nitric oxide synthase to cluster these molecules at synaptic junctions.
Table 10.31.
Scaffold proteins — Disc large homologs — members of the PSD95 family of the MAGUK superfamily. Members of the MAGUK superfamily intervene in the maintenence of the structure of submembrane domains, especially cell junctions, and can thus participate in signaling at these membrane regions.
Member | Aliases |
---|---|
DLg1 | SAP97 |
DLg2 | PSD93 |
DLg3 | SAP102 |
DLg4 | PSD95, SAP90 |
Guanylate kinase-associated protein (GKAP), or Disc large homolog-associated protein-1 (DLgAP1), binds directly to the 4 Disc large homologs, as well as SH3 and multiple ankyrin repeat domains protein SHAnk2,140 8-kDa cytoplasmic dynein light chain LC8 type 1 (DynLL1),141 and LC8 type 2 (DynLL2)142 to constitute molecular scaffolds in postsynaptic densities of neurons.143
Disc large homolog-associated proteins (Table 10.32) function in the transfer of DLg4 from the cytosol to the plasma membrane and may anchor molecules to facilitate interaction between postsynaptic density components, thereby being involved in signaling at neuronal postsynaptic densities [1282].
Table 10.32.
Disc large homolog-associated proteins ( GKAP: guanylate kinase-associated protein; SAPAP: SAP90/PSD95 (DLg4)-associated protein).
Type | Other Aliases |
---|---|
DLgAP1 | SAPAP1, DAP1, GKAP |
DLgAP2 | SAPAP2, DAP2 |
DLgAP3 | SAPAP3, DAP3 |
DLgAP4 | SAPAP4, DAP4, DLP4 |
The SH3 and multiple ankyrin repeat domain-containing protein SHAnk2144 abounds in postsynaptic densities. Members of the SHANK family that function as scaffolds in postsynaptic densities interact with synaptic proteins of the SAPAP–GKAP family. In particular, they can link DLg4 to plasmalemmal receptors and the cytoskeleton at glutamatergic synapses of the central nervous system [1283]. The SHAnk proteins connects to GKAP glutamate receptor-binding protein (GRIP), and Homer, thereby bridging the SHAnk–GKAP–DLg4–NMDAR, SHAnk–GRIP–AMDAR, and SHAnk–Homer–mGluR complexes in synapses [1284, 1285]. Alternative splicing of SHANK family members may regulate the spectrum of their interactors.
Table 10.33.
The SHANK family of scaffold proteins that serve as assembly platforms for other postsynaptic density proteins (CortBP: cortactin-binding protein ProSAP: Proline-rich synapse-associated protein SHAnk: SH3 and multiple ankyrin repeat-containing protein; SstRIP: somatostatin receptor-interacting protein).
Subtype | Other aliases |
---|---|
SHAnk1 | SPAnk1, SstRIP, synamon |
SHAnk2 | SPAnk3, ProSAP1, CortBP1 |
SHAnk3 | SPAnk2, ProSAP2 |
Synaptic cell adhesion molecules are implicated in the regulation of initial contacts between dendrites and axons, synapse formation and maturation, maintenance, and remodeling of established synapses. Adhesion molecules, such as neuroligins, neurexins, cell adhesion molecules (CAdM1–CAdM4; Table 10.34),145 netrin-G ligands (NGL1–NGL3),146 PTPRf, leucine rich repeat-containing transmembrane neuronal proteins (LRRTM1–LRRTM4), and EPHb receptors, can induce pre- and postsynaptic differentiation in contacting axons and dendrites, respectively. Synaptic cell adhesion molecules regulate synapse formation and structure via trans-synaptic and heterophilic adhesions.
Table 10.34.
Synaptic cell adhesion molecules (BIgR: brain immunoglobulin receptor; CadM: cell adhesion molecule; IGSF: immunoglobulin superfamily member; LRRC4: leucine rich repeat-containing protein-4; LRRTM: leucine rich repeat-containing, transmembrane, neuronal protein; NecL: nectin-like protein; NGLi: netrin-Gi ligand; SynCAM: synaptic cell adhesion molecule).
Subtype | Other aliases |
---|---|
CadM1 | SynCAM1, NecL2, IGSF4a |
CadM2 | SynCAM2, NecL3, IGSF4d |
CadM3 | SynCAM3, NecL1, IGSF4b, BIgR |
CadM4 | SynCAM4, NecL4, IGSF4c |
LRRC4a | NGL2 |
LRRC4b | NGL3 |
LRRC4c | NGL1 |
LRRTM1 | |
LRRTM2 | LRRN2 |
LRRTM3 | |
LRRTM4 |
10.8.4 A-Kinase-Anchoring Proteins
A-kinase-anchoring proteins147 are signal-organizing molecules that target protein kinases and phosphatases to subcellular loci where these enzymes control the phosphorylation state of neighboring substrates [1287]. A-kinase-anchoring proteins actually bind to diverse enzymes, not only protein kinase-A148 but also protein kinase-C, protein phosphatases, and phosphodiesterases [1288].
Signaling and scaffolding AKAP proteins coordinate different signaling enzymes according to the binding partner. In other words, AKAP proteins organize clusters of signaling mediators, thereby allowing signal segregation.
10.8.4.1 AKAP Family
A-kinase-anchoring proteins constitute a family of structurally diverse proteins (AKAP1–AKAP14; Table 10.35) that, in addition to protein kinase-A and -C, bind P21-activated kinase, phosphodiesterases, and phosphatases, such as PP1, PP2, and PP3. Alternative splicing generates transcript variants that encode different isoforms.
Table 10.35.
A-kinase-anchoring proteins (AKAP; AKAP-Lbc: AKAP-lymphoid blast crisis; BRx: breast cancer nuclear receptor-binding auxiliary protein; CG-NAP: centrosome- and Golgi-localized PKN-associated protein; D-AKAP: dual-specific AKAP; mAKAP: muscle-selective AKAP; PrKA: protein kinase A-anchoring protein; sAKAP: spermatid AKAP). A-kinase-anchoring proteins (PKA-anchoring molecules) contain a motif that binds to the N-terminal dimerization domain of the R subunit of protein kinase-A. Most AKAPs interact specifically with the PKA type-2 regulatory (R2) subunit (i.e., PKA2), but several R1-specific AKAPs exist. Dual-specificity D-AKAPs interact with both type-1 and -2 regulatory subunits (of PKA1 and PKA2, respectively).
Type | Other aliases and names |
---|---|
AKAP1 | AKAP84, AKAP121, AKAP149, D-AKAP1, sAKAP84 |
AKAP2 | AKAPkl |
AKAP3 | AKAP110 |
AKAP4 | AKAP82, FSC1 |
AKAP5 | AKAP75, AKAP79, AKAP150 |
AKAP6 | AKAP100, mAKAP, PrKA6 |
AKAP7 | AKAP15, AKAP18 |
AKAP8 | AKAP95 |
AKAP9 | AKAP350, AKAP450, CG-NAP, PrKA9, hyperion, yotiao |
AKAP10 | D-AKAP2 |
AKAP11 | AKAP220 |
AKAP12 | AKAP250, gravin |
AKAP13 | AKAP-Lbc, Ht31, BRx, ARHGEF13 (RhoGEF13) |
AKAP14 | AKAP28 |
Some AKAPs are able to bind both PKA type-1 and -2 regulatory subunits (dual-specific AKAP1 and AKAP10). Both AKAP1 and AKAP10 are mitochondrial; AKAP3 and AKAP4 are linked to sperm; AKAP5 is predominantly expressed in the cerebral cortex, but also in T lymphocytes; AKAP6 anchors PKA to the nuclear envelope and sarcoplasmic reticulum, especially in the central nervous system, skeletal muscles, and myocardium; AKAP8 has a cycle-dependent interaction with PKA; AKAP9 localizes to the Golgi body and centrosome; AKAP11 to testis; AKAP12 to endothelial cells. Protein AKAP13 serves as guanine nucleotide-exchange factor for the RHO superfamily of small GTPases (RhoGEF13).
10.8.4.2 Spatiotemporal Regulation of Cell Signaling
A-kinase-anchoring proteins contribute to the spatial regulation of signaling events, targeting the enzyme to specific sites (plasma membrane, mitochondria, cytoskeleton, or centrosome), where they localize, as different AKAP types can have specific cytoplasmic distribution.
Within a given site, a given AKAP can link to diverse substrates and different AKAPs can assemble distinct signaling complexes.149 The displacement of enzymes into and out of these complexes contributes to the temporal regulation of signaling events.
Members of the AKAP family form complexes with enzymes that trigger the following step of signal transduction or terminate signaling. These site then regulate the forward and backward steps of a given signaling process.
The location of an AKAP complex can be modulated by competition between binding partners, for example, between an enzyme and a component of the cytoskeleton. It can also be modulated by AKAP phosphorylation. The recruitment or release of AKAP-binding partners can alter the response to signals or change the location of a signaling complex to yield a dynamic localization and reorganization of AKAP complexes.
Compartmentation of AKAP–PKA complexes relies on specialized targeting domains of each anchoring protein, the so-called localization signals. Several AKAPs can lodge at the same subcellular compartment. On the other hand, alternatively spliced variants can be assigned to different regions [1290].
Protein AKAP5 anchors to plasmalemmal phospholipids; AKAP12 also tethers to the plasma membrane using an N-terminal myristoyl group and phospholipid-binding sequences (Tables 10.36 to 10.38). Recruitment to membranes of AKAP7α and AKAP7β relies on myristoyl and palmitoyl groups. Protein AKAP9 targets the centrosome using the pericentrin-AKAP9 centrosomal-targeting domain (PACT).150
Table 10.36.
Examples of AKAP-based complexes that create focal points for signal transduction (Sources: [1290, 1291]). Numerous AKAPs target PKA to the plasma membrane, cytoskeleton, endoplasmic reticulum, Golgi body, mitochondria, perinuclear region, and nuclear matrix. The AKAP scaffolds not only anchor PKA, but also assemble multienzyme signaling complexes that ensure the integration and processing of diverse signaling pathways. They indeed coordinate the activity of many kinases, phosphatases, phosphodiesterases, adenylate cyclases, GTPases, and other regulators.
Type | Partners |
---|---|
Plasma membrane | |
AKAP5 | PKA, PKC, PP3, AMPAR, NMDAR, DLg1/4 (neuron); |
PKA, PKC, PP3, AC5, AC6, Cav3, β2AR, | |
CaV1.2 (cardiomyocyte | |
CaV1.2, KV7.2, Aqp | |
AKAP7 | PKA, CaV1.2, NaV |
AKAP9 | PKA, CK1, PP1, NMDAR, KV7.1, IP3R |
AKAP12 | PKA, PKC, β2AR |
Perinuclear region | |
AKAP6 | PKA, PDE4d3 |
Endo(sarco)plasmic reticulum | |
AKAP6 | PKA, RyR2 |
Centrosome, Golgi body | |
AKAP9 | PKA, PKA, PKN, PP1, PP3, PDE4d3, GCP2/3 |
ClIC | |
Actin cytoskeleton | |
AKAP12 | PKA, PKC |
AKAP13 | PKA, PKC, PKD, Rho, 14-3-3 |
Mitochondrion | |
AKAP1 | PKA, PP1 |
Peroxisomes | |
AKAP11 | PKA, PP1, GSK3β |
Table 10.37.
Cardiac AKAPs (Part 1; Source: [1291]; AC: adenylate cyclase; AR, adrenoceptor; ERK: extracellular signal-regulated kinase; HIF: hypoxia-inducible factor; NCX: sodium–calcium exchanger; MyCBP: Myc-binding protein; NFAT: nuclear factor of activated T cells; PDE: phosphodiesterase; PDK: phosphoinositide-dependent kinase; PKA/C/D/N: protein kinase-A/C/D/N; PP: protein phosphatase; PTP: protein Tyr phosphatase; RyR: ryanodine receptor; SIAH: seven in absentia homolog [Ub ligase]; VHL: von Hippel-Lindau ubiquitine ligase). In cardiomyocytes, cAMP messenger transmits signals sent by β2-adrenergic receptors of sympathetic nerves via catecholamines (noradrenaline and adrenaline). It activates PKA to enhance cardiac inotropy, chronotropy, and lusitropy. The cAMP–PKA axis leads to phosphorylation (opening) of CaV1.2 and RyR2 channels. In addition, phosphorylation of phospholamban that inhibits SERCA2 causes the dissociation of PLb from SERCA2, thereby activating SERCA2 for relaxation. Phosphorylation of sarcomeric troponin-I and myosin-binding protein-C also promotes relaxation by decreasing Ca
responsiveness of myofilaments. Under prolonged catecholaminergic stimulation, cAMP contributes to cardiomyocyte hypertrophy and apoptosis.

Type | Location | Binding partners | Function |
---|---|---|---|
AKAP1 | Mitochondria, | PKA1/2, PKCα, | Regulation of |
nuclear envelope, | Src, RSK1, PP1/3, | cardiomyocyte | |
endoplasmic reticulum | PTPn21, PDE7a, | hypertrophy | |
RhoGEF2, MyCBP | |||
AKAP5 | Plasma membrane, | PKA2, PKC, PP3, AC5/6 | Calcium |
T tubules | KV7.2, CaV1.2, | signaling | |
βAR, DLg1, Cav3 | |||
AKAP6 | Nuclear envelope | PKA2, PDK1, RSK3, | Regulation of |
MAP2K5, ERK5, PP2/3, | CMC hypertrophy, | ||
PDE4d3, AC5, | HIF1α stability, | ||
RyR2, NCX1, NFAT, | calcium signaling | ||
Rap1, RapGEF3, | |||
HIF1α, VHL, SIAH2, | |||
nesprin-1α, | |||
synaptopodin-2 | |||
AKAP7 | Plasma membrane, | PKA2, PP1, | Calcium signaling |
endoplasmic reticulum | CaV1.2, | ||
phospholamban, | |||
inhibitor-1 |
Table 10.38.
Cardiac AKAPs (Part 2; Source: [1291]; BCR, breakpoint cluster region protein [GAP]; ClIC: chloride intracellular channel; ERK: extracellular signal-regulated kinase; IP3R: inositol trisphosphate receptor; KSR: kinase suppressor of Ras; NHERF3: sodium–proton exchange regulatory factor-3 [a.k.a. CFTR-associated protein CAP70 and PDZ domain-containing protein PDZK1]; PDE: phosphodiesterase; PI3K: phosphatidylinositol 3-kinase; PKA/C/D/N: protein kinase-A/C/D/N).
Type | Location | Binding partners | Function |
---|---|---|---|
AKAP9 | Plasma membrane, | PKA2, PKCε, PKN1, | Cardiac |
Golgi body, | CK1, ACase, PDE4d3, | repolarization | |
centrosome | IP3R, KV7.1, ClIC | ||
AKAP10 | Outer mitochondrial | PKA1/2, NHERF3, | Cardiac |
membrane | Rab4/11 | rhythm | |
AKAP13 | Cytoskeleton | PKA2, PKCη, PKD, | Regulation of |
Raf, MAP3K15, MAP2K1–3, | CMC | ||
ERK1/2, P38MAPKα, | hypertrophy | ||
PKNα, PI3K, Ras, KSR1, | |||
PDE3b, G12, Gβγ, | |||
RhoA, BCR, 14-3-3 |
10.8.4.3 AKAP Post-Translational Modifications
The location of AKAP complexes can reorganize. The recruitment or release of AKAP-binding partners influences the response to transmitted signals. Competition between binding partners results from mutually exclusive binding to AKAP proteins [1290]. In addition, modification (e.g., phosphorylation, myristoylation, and palmitoylation) of the AKAP localization signal affects the location of AKAP scaffolds.
Regulatory phosphorylation can constitute feedback loops. Dynamic regulation of PKA activity relies on antagonist component of AKAP-based complexes, such as cAMP activator and its inhibitor phosphodiesterase that can both anchor to AKAP protein. Whereas hormonal stimulation increases the cAMP concentration, hence enabling PKA activation, recruited phosphodiesterase prevents cAMP action, thereby repressing PKA activation (negative feedback).
Recruitment and release of AKAP-binding partners is regulated by phosphorylation. For example, phosphorylation-dependent recruitment of 14-3-3 protein suppresses the RhoGEF activity of AKAP13 agent. On the other hand, phosphorylation of AKAP13 at another site precludes its association with protein kinase-D [1290]. Recruitment and release of binding partners to and from AKAP12 are also controlled by phosphorylation.
AKAP1
Protein AKAP1 and its shorter splice variant AKAP1S are widespread mitochondrial proteins. In ventriculomyocytes, AKAP1 precludes maladaptive hypertrophy [1291]. It actually hinders dephosphorylation and nuclear translocation of the prohypertrophic NFAT3 transcription factor.
AKAP2
Protein AKAP2 lodges in the lung, kidney, and cerebellum [1292]. Different isoforms predominate in different tissues. Anchor AKAP2 has a polarized distribution, as it accumulates near the apical surface of the epithelium in nephron tubules. It also abounds in alveolar epithelial cells, below the plasma membrane. It interacts with and modulates the structure of the actin cytoskeleton.
AKAP3 and AKAP4
Protein AKAP3 is expressed in the testis, but not in other tissues. Protein AKAP4 resides in the fibrous sheath of the sperm flagellum, a unique cytoskeletal structure. The AKAP4 mature form can bind AKAP3 protein. The latter is involved in the organization of the basic structure of the fibrous sheath, whereas AKAP4 intervenes in completing fibrous sheath assembly [1293].
AKAP5
Neuronal AKAP5 anchors not only the cAMP-dependent kinase PKA, but also Ca
– and lipid-regulated PKC, and calmodulin-stimulated protein phosphatase PP3.

In cardiomyocytes, AKAP5 assembles a signaling complex that contains β-adrenoceptors, adenylate cyclases AC5 and AC6, PKA, PP3, caveolin-3, andCaV1.2 channels that are phosphorylated upon sympathetic stimulation [1291]. In fact, the AKAP5 complex recruits AC5 and AC6 and produces cAMP in nanodomains, where PKA processes not only CaV1.2 channels, phospholamban, and RyR2 receptors located in caveolin-3-associated junctional regions of the sarcoplasmic reticulum adjacent to T tubules (Vol. 5 – Chap. 5. Cardiomyocytes).
In dendrites of excitatory synapses, AKAP5 forms a postsynaptic signaling complex with PKA and PP3 enzymes as well as GluR1 subunit of the AMPA-type glutamate receptor via DLg1 or DLg4 adaptor [1290]. Anchored PKA phosphorylates (activates) GluR1 (Ser845), whereas anchored PP3 dephosphorylates this site, thereby activating DLg4-associated double minute-2 ubiquitin ligase. The latter ubiquitinates DLg4 for proteasomal degradation and enables internalization of AMPA receptors.
In fact, neuronal AKAP5 can bind to different types of ion channels: (1) ionotropic AMPA-type glutamate receptors (iGluR) that propagate excitatory stimuli via interaction with membrane-associated guanylate kinase (MAGuK) scaffold protein Disc large homolog DLg1 and (2) M-typeKV7.2 and KV7.3 channels. Ligand-gated ion channel iGluR requires anchoring of PKA and PP3 for full activity. Protein kinase-A is necessary to maintain AMPAGlu channels at the plasma membrane. Protein phosphatase-3 is needed to lower AMPA activity in response to tonic stimulation. Protein AKAP5 regulates activities of these different enzymes and channels in hippocampal (PKA and PP3 are active; PKC is inactive in this subset of excitatory synapses) and superior cervical ganglion neurons of the sympathetic nervous system (PKA and PP3 remain inactive; PKC is active), respectively [1294]. The AKAP5–GluR complex brings PKA kinase and PP3 phosphatase in proximity to the channel to control its phosphorylation state. (PKA activates, whereas PP3 inactivates). On the other hand, the AKAP5–KV7.2 (3)–PKC complex inhibits KV7.2 and KV7.3 channels, thereby attenuating the hyperpolarizing K + flux in response to exciting signals from M1 muscarinic receptors. Muscarinic suppression of M currents proceeds via Gq/11-coupled signaling requires Ca
, phosphatidylinositol (4,5)-bisphosphate, and diacylglycerol, as well as phosphorylation by AKAP5-bound PKC enzyme.

AKAP6
Muscle-specific A-kinase-anchoring protein AKAP6 complexes with PKA, phosphodiesterase PDE4d3 and the guanine nucleotide-exchange factors for GTPase Rap1 RapGEF3 [1295].
In cardiomyocytes, assembling occurs at the perinuclear membrane. Phosphodiesterase-D3 serves as an adaptor for RapGEF3 and ERK5 proteins. Phosphorylation of PDE4d3 by AKAP6-associated ERK favors cAMP production and subsequent PKA and RapGEF3 activation. However, subsequent phosphorylation of PDE4d3 by PKA reduces cAMP concentration, thereby causing PKA deactivation and suppressing RapGEF3 excitation of AKAP6-associated ERK5 activity. The latter provokes cardiomyocyte hypertrophy.
Scaffold AKAP6 directly binds RyR2 channels. It may favor RyR2 phosphorylation by PKA and subsequent release of the inhibitor 12.6-kDa FK506-binding protein FKBP1b to increase RyR2 open probability. The RyR2–AKAP6 complex may localize either to the entire sarcoplasmic reticulum or only to a perinuclear compartment [1291]. The AKAP6 complex only permits a transient activation of PKA in response to βAR stimulation.
In cardiomyocytes, AKAP6β localizes to the outer nuclear membrane, as it interacts with nesprin-1α, which binds to actin filaments [1291].151 Scaffold AKAP6β assembles a complex with PKA, ERK5, PP3, AC5, PDE4d3, RyR2, NFAT3, and RapGEF3 proteins. Activated PKA phosphorylates (inactivates) adenylate cyclase AC5 and (activates) phosphodiesterase PDE4d3, thereby decreasing cAMP synthesis and increasing cAMP degradation (negative feedback loop).
Anchored PKA also fosters PP2 activity that dephosphorylates PDE4d3 and RyR2, thereby enhancing Ca
influx from intracellular stores. In addition, PDE4d3 can also act as a scaffold that recruits the cAMP-activated guanine nucleotide-exchange factor RapGEF3, prohypertrophic mitogen-activated protein kinase kinase MAP2K5, and ERK5 to the AKAP6 complex. Upon elevation of local cAMP concentration that stimulates RapGEF3, activated Rap1 inhibits the ERK5 axis. The latter kinase impedes PDE4d3 action, thereby repressing cAMP degradation.

The AKAP6 complex integrates hypertrophic signals initiated by α1- and β-adrenergic receptors, endothelin-1 receptors, leukemia inhibitor factor receptors, phosphatase PP3 catalytic subunit isoform PP3cβ, and NFAT3 transcription factor. In response to adrenoceptor activation, anchored PP3cβ dephosphorylates (activates) NFAT3 that supports the transcription of hypertrophic genes. Scaffold AKAP6β also binds phospholipase-Cε that assists endothelin-1-mediated hypertrophy [1291].
Protein AKAP6 assembles a signaling complex with hypoxia-inducible factor HIF1α, prolyl hydroxylase domain-containing proteins (PHD),152 and ubiquitin–protein ligases von Hippel-Lindau protein and Seven in absentia homolog SIAH2 that ubiquitinate HIF and PHD, respectively, for proteasomal degradation [1291]. This AKAP6 complex thus serves as a regulator of cell response to hypoxia.
AKAP7
Four isoforms (AKAP18α–AKAP18δ) have been identified. Subtype AKAP18δ anchors and controls β-adrenergic regulation of SERCA2 activity [1291]. It indeed favors PKA-mediated phosphorylation of phospholamban, thereby dissociating phospholamban from SERCA2 and fostering Ca
reuptake into the sarcoplasmic reticulum and cardiomyocyte relaxation.153

In addition, AKAP18δ recruits protein phosphatase PP1 and PP1r1c inhibitory subunit. Phosphorylation by PKA of PP1r1c prevents PP1 activity [1291].
AKAP8
In mammalian cells, AKAP8 localizes exclusively to the nuclear matrix. DEAD (Asp-Glu-Ala-Asp) box protein DDx5, or RNA helicase P68, is a binding partner of AKAP8 [1296]. Protein AKAP8 strongly interacts with the 3 cyclins-D, but not with cyclin-dependent kinase CDK4 [1297]. It also directly interacts with minichromosome maintenance protein MCM2, a component of the hexameric MCM2–MCM7 DNA helicase prereplication complex.
AKAP9
In cardiomyocytes, AKAP9 recruits an enzymatic complex made of a kinase (PKA), a phosphatase, and a phosphodiesterase (PDE4d3), to fine tune slow-activating, delayed rectifier K + current (i Ks) throughKV7 channels that contributes to the repolarization of the plasma membrane of cardiomyocytes [1291]. In response to β-AR stimulation, PKA phosphorylates KV7.1 (Ser27) to augment K + efflux, accelerates repolarization, and shortens action potential duration. Maximal activation of KV7 by the sympathetic system occurs when anchored PKA also phosphorylates AKAP9 (Ser43). In addition, AKAP9 links to chloride intracellular channel (ClIC).
AKAP10
Scaffold AKAP10 is involved in the regulation of the cardiac rhythm [1291]. This dual-specific AKAP that targets both PKA1 and PKA2 abounds in mitochondria. It contains regulator of G-protein signaling domains, in addition to a PKAR2 subunit-binding domain.
In the kidney, many plasmalemmal transporters of the apical brush border of proximal tubular cells ensure the maintenance of electrolyte concentrations. Sodium–phosphate cotransporter-2, or SLC34a1, interacts with 7 types of PDZ domain-containing proteins, among which sodium–hydrogen exchanger regulatory factors NHERF1 (SLC9a3R1) and NHERF3 (PDZK1) [1298]. Factor NHERF3 also links to solute carrier SLC17a1, or sodium–phosphate cotransporter-1, Na + –H + exchanger NHE3 (SLC9a3), organic cation transporter OCTN1 (SLC22a4), chloride–formate exchanger (CFEx or SLC26a6), and urate–anion exchanger Urat1 (SLC22a12), in addition to Ste20-like kinase SLK and AKAP10, in the brush border of proximal tubular cells.
AKAP11
Glycogen synthase kinase GSK3β binds to AKAP11, in addition to cAMP-dependent protein kinase-A and protein phosphatase PP1 [1299]. Kinase PKA phosphorylates (inactivates) AKAP11-anchored GSK3β, more efficiently than free GSK3β. On the other hand, PP1 dephosphorylates GSK3β.
AKAP12
Two major isoforms (AKAP12α–AKAP12β) are created by independent promoters. They are synthesized in most mesechymal cells, some epithelial cell types, endothelial cells, and some specialized cells, such as podocytes, Purkinje cells, and astrocytes [1300]. A shorter third isoform, AKAP12γ, is specific to the testis.
Scaffold AKAP12 binds to PKC, Src kinase, calmodulin, cyclins, Factin, a membrane-associated form of (1,4)-galactosyltransferase associated with adhesion signaling, and phosphoinositols [1300].
Protein AKAP12 abounds at inner plasma membrane sites, along the cortical cytoskeleton, and in the perinuclear region.
At the neuromuscular junction, recruitment and release of binding partners to AKAP12 depend on phosphorylation by PKA and PKC kinases. The AKAP12 complex is recruited to the plasma membrane by interacting with β2-adrenoceptor. Agonist stimulation initially strengthens the association of AKAP12 with β2AR, upon AKAP12 phosphorylation by PKA kinase [1290]. Prolonged agonist stimulation dismantles the AKAP12 complex, upon phosphorylation by PKC that primes ubiquitination by DM2 ligase, which is recruited by adaptor β-arrestin, for proteasomal degradation.
AKAP13
Regulator AKAP13 is a guanine nucleotide (GDP-to-GTP)-exchange factor that links G12/13 subclass subunits to Rho activation [1290]. Dimerizing 14-3-3 polypeptides bind to specific motifs with phosphorylated Ser or Thr residues. Phosphorylation by PKA of AKAP13 enables the recruitment of 14-3-3 proteins, thereby preventing the AKAP13 RhoGEF activity.
In cardiomyocytes, AKAP13 acts as a PKA2 anchor and RhoAGEF protein. Its activity is fostered by Gq-coupled α1-adrenoceptors and G12-coupled endothelin-1 receptor. On the other hand, AKAP13-anchored PKA limits AKAP13 activity, thereby lowering RhoA activation via the recruitment of 14-3-3 protein [1291].
Scaffold AKAP13 can form a signaling complex with the scaffold kinase suppressor of Ras KSR1, and Raf, MAP2K1, and MAP2K2 kinase, that excites ERK1 and ERK2 kinases [1291].
Protein AKAP13 mediates the hypertrophic response induced by several G-protein-coupled receptors, such as α1-adrenoceptors, AT1 angiotensin-2 receptor, and endothelin-1 receptor [1291]. Scaffold AKAP13 recruits protein kinase-D and its upstream activator protein kinase-Cη. Stimulation of α1-adrenoceptors or endothelin-1 receptors excites anchored PKCη that phosphorylates (activates) AKAP13-bound PKD enzyme [1291]. Nuclear PKD activity that is enhanced by AKAP13 leads to phosphorylation and nuclear export of class-2 histone deacetylase HDAC5, thereby supporting myocyte-specific enhancer-binding factor-2-dependent transcriptional activation of hypertrophic genes. On the other hand, active PKD is released from AKAP13 when this scaffold is phosphorylated by anchored PKA kinase.
AKAP14
Mucociliary clearance relies on the coordinated beating of cilia that line the wetted surface of the airway epithelium in the respiratory tract. Ciliary beat results from the coupling between ATP hydrolysis by dynein, the axonemal nanomotor, to microtubule sliding. Activated axonemal protein kinase-A increases the ciliary beat frequency in humans. In ciliary axonemes, AKAP14 anchors PKA holoenzyme in the axoneme, close to its substrates [1301].
10.8.5 Annexins
Annexins154 are Ca
-regulated proteins and Ca
sensors.155 These Ca
effectors are organizers of membrane domains and membrane-recruitment platforms for proteins with which they interact. Annexins mediate cellular responses to changes in the intracellular Ca
concentration.




10.8.5.1 Annexin Family
Annexins constitute a family of proteins that belongs to the set of EF-hand proteins.156 Proteins of the annexin family thus share the ability to link to both Ca
and membrane lipids.157

Five major annexin subclasses (A–E) are defined with vertebrate (human) annexins (A1–A13) encoded by the genes ANXA1 to ANXA11 and ANXA13. Animals, but not humans, possess members of the annexin-B set [1302]. Annexins-C, -D, and -E are observed in fungi, plants, and protists, respectively.
10.8.5.2 Subcellular Localization
Soluble annexins are distributed in the cytoplasm at a resting Ca
concentration. Several factors influence annexin transport and localization in cells.

In fibroblasts, annexin-A1, -A4, and -A5 are observed in the nucleus with higher concentrations than in the cytoplasm [1303]. Extranuclear annexin-A4 partly localizes to the endoplasmic reticulum. Annexin-A1 and -A2 lodge in the plasma membrane. When the intracellular calcium concentration rises, intranuclear annexin-A4 and -A5 relocalize to the nuclear envelope, whereas the cytosolic pool of these annexins is situated at the plasma membrane; plasmalemmal annexin-A2 follows a punctate pattern, although it has a homogeneous distribution before calcium stimulation; annexin-A6 relocalizes to the plasma membrane with a more homogeneous distribution.
10.8.5.3 Structural and Functional Features
Annexins operate on actin polymerization at cellular membranes, structuring of endosomal compartments, intracellular Ca
-regulated transfer, and midbody formation during cytokinesis [1304]. Annexins are then involved in membrane–cytoskeleton attachments, endo- and exocytosis, and regulation of ion fluxes across membranes.

Structure–Function Relationship
Annexins contain the annexin core domain, a Ca
– and membrane-binding module. This core domain enables Ca
-bound annexins to dock onto membranes that contain negatively charged phospholipids [1304]. Most annexins can then interact with cellular membranes in a reversible and controlled fashion.


Phosphatidylserine, phosphatidylinositol, and phosphatidic acid are among the preferred binding acidic phospholipids. Some annexin types interact more specifically with certain membrane lipids: annexin-A2 binds to phosphatidylinositol (4,5)-bisphosphate; annexin-A3, -A4, -A5, and -A6 to phosphatidylethanolamine; and annexin-A5 to phosphatidylcholine [1304].
Annexins can tether to 2 membranes. This feature is influenced by phosphorylation of their N-terminus. Simultaneous linkage to 2 cell membrane of annexin-A1, -A2, and -A4 is inhibited by phosphorylation, whereas that of annexin-A7 is promoted [1304]. On the other hand, phosphorylation do not strongly affects the ability of annexins to bind to a single membrane.
Calcium concentration that initiate phospholipid binding differ markedly between annexin types. It ranges from 20 μmol for the connection between annexin-A5 and phosphatidylserine of liposomes to submicromolar Ca
concentrations for annexin-A1 and -A2 to phosphatidylserine and phosphatidic acid and less than 100 nmol for the annexin-A2–S100a10 complex to phosphatidylserine [1304].

Calcium-independent membrane binding depends on the pH value. For example, annexin-A5 binds to and apparently penetrates the bilayer of phosphatidylserine vesicles at pH 4 in the absence of Ca
ions, whereas, at neutral pH, Ca
binding enables lipid interaction [1302].


Annexin also possess a N-terminal interaction domain with binding sites for cytoplasmic proteic ligands, which can then be recruited to membranes via the annexin core-mediated phospholipid interaction. Once annexin is attached to a membrane, its cytoplasmic face can connect to proteins, thereby assembling these interactors at membrane sites in a Ca
-regulated manner.

Cell Export and Import
Membrane-bound annexins can form self-assemblies that influence the mobility and organization of membrane lipids. They can then participate in the regulation of organization of membrane domain, in particular, those involved in material tranfer.
Exocytosis
Externalization of proteins, polypeptide hormones, and amine neurotransmitters terminates with the fusion of secretory vesicle membranes with the plasma membrane. Mature secretory vesicles can accumulate within secretory cells, waiting for a stimulus-triggered Ca
influx. Upon Ca
entry, vSNAREs and tSNAREs intertwine with one another and rearrange the lipid bilayers for fusion (Vol. 1 – Chap. 9. Intracellular Transport). Calcium-binding proteins lodge on membranes and in the cytoplasm of secretory cells to participate in exocytosis, such as EF-hand proteins (calmodulin and S100 proteins) and C2 domain-containing proteins (synaptotagmin, rabphilin, Rab3-interacting molecule, and MUnc13), in addition to annexins.


Annexin-A2 is a component of filamentous actin that carry newly formed endocytic vesicles from the plasma membrane into the cytoplasm. The activity of annexin-A2 in exocytosis depends on its phosphorylation of its N-terminus by protein kinase-C [1304]. Annexin-A2 and -A7 mediates aggregation and apparent fusion of hormone-containing chromaffin granules of neuroendocrine cells in the medulla of the adrenal gland [1305]. Annexin-A1 promotes the calcium-dependent fusion of liposomes as well as fusion of phospholipid vesicles with plasma membranes in neutrophils.
Exocytosis
Several annexins, such as annexin-A1, -A2, and -A6, reside in endosomal compartments. In particular, annexin-A2 intervenes in the lysosomal transport and endocytosis of EGF receptors. Two sequential steps of multivesicular endosome genesis exist. The first step require hepatocyte growth factor-regulated Tyr kinase substrate (HRS) and endosomal sorting complex required for transport proteins (ESCRT) for receptor sorting and inward vesiculation. The second step relies on annexin-A2 on early endosomes that facilitates the detachment of multivesicular regions of early endosomes [1304].
The annexin-A2–S100a10 complex also contributes to the morphology of perinuclear Rab11 + recycling endosomes [1304]. In addition, annexin-A2 composes the chaperone complex with caveolin-1 and cholesteryl esters that carries cholesteryl esters from caveolae to internal membranes.
Annexin-A1 is involved in the inward vesiculation in multivesicular endosomes Annexin-A6 is required in cysteine peptidase-dependent budding of clathrin-coated pits.
Ion Flux
Annexin-A2, -A4, and -A6 modulate plasmalemmal Cl − channels and sarcoplasmic reticulum Ca
channels. In association with S100a10, annexin-A2 interacts with Na + channel, 2-pore acid-sensitive K + channel-1 (TASK1), and Ca
TRPV channels (TRPV5 and TRPV6) [1304].


Extracellular Activity
Certain annexins not only act inside the cell, but also have extracellular functions, once they are released from the cell. Annexin-A1 inhibits leukocyte extravasation. On the vascular endothelium, annexin-A2 is a coreceptor for tissue plasminogen activator and plasminogen, inducing fibrinolysis. Annexin-A5 may mask the membrane phospholipids used by coagulation factors in the clotting cascade.
10.8.5.4 Partners
Once bound to certain cell membrane phospholipids via membrane-binding domain owing to calcium ions (Ca
-dependent linkage),158 annexins interact with proteins (Table 10.39) and provide Ca
signaling. Many cytosolic ligands pertain to the EF-hand Ca
-binding proteins, especially S100 proteins.159



Table 10.39.
Annexins (Anx) and their partners (CAP: calcyclin-associated protein; Cal: calpactin; Cal1H: calpactin-1 heavy chain; Cal1L: calpactin-1 light chain; Cpb: calphobindin; Cbd: chromobindin; ClCa: Ca
-activated Cl − channel; Enx: endonexin; GAG: glycosaminoglycan; IP2: inositol (1,2)-bisphosphate; ISA: intestine-specific annexin: Lpc: lipocortin; Maxi: Maxi chloride channel; PAP: placental anticoagulant protein; Ppx: pemphaxin; Snx: synexin; VAC: vascular anticoagulant).

Type | Aliases | Partners |
---|---|---|
AnxA1 | Lpc1, Cal2, | PI(4,5)P2, cPLA2, actin, |
Cbd9 | S100a10 (Cal1L), S100a11, EGFR, ATP, | |
purine-rich RNA, pyrimidine-rich DNA | ||
AnxA2 | Lpc2, Cal1H, | PI(4,5)P2, cholesteryl esters, caveolin-1, |
Cbd8, PAP4 | S100a10, Cl − channel, TASK1, TRPV5/6, | |
GAGs, RNA | ||
AnxA3 | Lpc3, PAP3 | IP2 |
AnxA4 | Lpc4, Enx1, | ClCa, |
Cbd4, PAP2 | GAGs | |
AnxA5 | Lpc5, Enx2, Cbp1 | CFTR, VEGFR2, |
Cbd4, PAP1 | GAGs | |
AnxA6 | Lpc6, Cpb2, | Maxi, GAGs, ATP, |
Cbd20 | dynamin, RasA1, Fyn, PYK2 | |
AnxA7 | Snx | Sorcin, GAGs, GTP |
AnxA9 | Anx31, Ppx | |
AnxA10 | Anx14 | |
AnxA11 | CAP50 | S100a6 |
AnxA13 | ISA | NEDD4 |
Annexins respond to an increase in Ca
concentration by translocating to membranes (both the plasma membrane and organelle membranes). Membrane-bound annexins acts as membrane scaffolds, forming protein clusters.

10.8.5.5 Annexin-A1
Annexin-A1160 may merge opposing membranes of the invaginating bud to close the neck of the nascent vesicle and enable scission from the plasma membrane. Moreover, annexin-A1 can act on vesicle fusion in the presence of Ca
. Stimulation by EGF of EGF receptor Tyr kinase leads to formation of a class of endosomes,161 EGFR-containing multivesicular endosomes, which requires phosphatidyl inositol 3-kinase and annexin-A1 [1306].162

Phospholipid-binding protein annexin-A1 can connect to cytosolic phospholipase-A2α (cPLA2α) at the Golgi body in endothelial cells [1307]. Cytosolic phospholipase-A2 cleaves phospholipids in a Ca
-dependent manner into arachidonic acid and lysophospholipid. The former is then converted into prostaglandins by cyclooxygenases and proper synthases.

Annexin-A1 can be phosphorylated by Tyr kinases to alter its Ca
sensitivity. Annexin-A1 can segregate lipids within the plasma membrane. It has a high affinity for PI(4,5)P2 to form raft-like domains rich in this lipid. Annexin-A1 binds to actin, thereby yielding attachment points where the cytoskeleton links to the plasma membrane. In addition, annexin-A1 can heteromerize with calcium-binding S100a10 and S100a11 proteins.163

Annexin-A1 can be exported from cells and intervene in inflammation. It hinders extravasation of neutrophils and monocytes. Extracellular annexin-A1 and/or its proteolytically removed N-terminus (NAnxA1) can bind to G-protein-coupled chemoattractant receptors of the formyl peptide receptor family (FPR and FPR-like receptors FPRL1 and FPRL2; Vol. 3 – Chap. 7. G-Protein-Coupled Receptors) [1304].
Annexin-A1 is also involved in apoptosis. Plasmalemmal annexin-A1 may be required for the clearance of apoptotic cells.
10.8.5.6 Annexin-A2
Annexin-A2164 is involved in cell motility, linkage of membrane-anchored protein complexes to the actin cytoskeleton, endo- and exocytosis, fibrinolysis, ion channel formation, and cell–matrix interactions. Annexin-A2 is a component of the Factin end, which propels newly formed endocytic vesicles from the membrane to the cytoplasm (actin-dependent transport). Annexin-A2 is also involved in membrane raft formation, especially in the smooth muscle cells. Annexin-A2 forms a complex with caveolin-1 and cholesteryl esters.
Like annexin-A1, annexin-A2 binds to PI(4,5)P2 and can segregate lipids within the plasma membrane and provide membrane attachment points for the cytoskeleton. The complex between annexin-A2 and S100a10 is implicated in the control of plasmalemmal Cl − channels, TRPV5 and TRPV6 channels, and acid-sensitive K + channel TASK1 (K2P3.1).
Annexin-A2 can be phosphorylated and, then, translocates into the nucleus, where it can bind to RNA.
The annexin-A2–S100a10 complex can localize to the surface of vascular endothelial cells, where it functions as a receptor for tissue plasminogen activator and plasminogen [1304]. Therefore, it supports the synthesis of plasmin that degrades fibrin. Furthermore, annexin-A2 controls Ca
-regulated exocytosis of von Willebrand factor and P-selectin.

10.8.5.7 Annexin-A3
Annexin-A3165 impedes the activity of phospholipase-A2. It is associated with acidic phospholipid-stimulated, Ca
– and Mg
-dependent inositol (1,2)-cyclic phosphate 2-phosphohydrolase that cleaves inositol (1,2)-bisphosphate into inositol 1-phosphate. It also operate as an anticoagulant.


Annexin-A3 abounds in neutrophils, whereas it is produced at low levels or is undetectable in many cell types. Annexin-A3 is associated with cytoplasmic granules in neutrophils and monocytes and translocates to the plasma membrane in activated cells [1305].
10.8.5.8 Annexin-A4
10.8.5.9 Annexin-A5
Like annexin-A2, annexin-A5167 can also enter into the nucleus upon tyrosine phosphorylation.
Annexin-A5 forms a shield around negatively charged phospholipids, thereby blocking the entry of phospholipids into blood coagulation.168
Annexin-A5 tethers to the Ca
– and cAMP-activated cystic fibrosis transmembrane conductance regulator (CFTR), a chloride channel [1311]. The function and localization of CFTR in normal epithelial cells of human bronchi depend on annexin-A5.

Apoptosis is characterized by an early change in cell volume, the so-called apoptotic volume decrease, especially in cardiomyocytes. The cell shrinkage results from the activity of volume-sensitive chloride channels [1313]. Annexin-A5 serves as an early markers of apoptosis. Agents PI3K, PKB, and ERK1 and ERK2 repress the activity of volume-sensitive chloride channels and protect against apoptosis [1314].
10.8.5.10 Annexin-A6
Annexin-A6169 possesses 2 Ca
-binding core domains that enables it to bind to different membrane regions. Alternative splicing gives rise to 2 isoforms. Annexin-A6 is involved in endosome aggregation and vesicle fusion during exocytosis in secreting epithelia.

Chloride is the main anion of extracellular fluid in the fetus and adult, but, at all gestational stages, fetal Cl − ion concentration is 5 to 6 mmol higher than in maternal blood. Maxi chloride channels lodge in secreting and absorbing epithelia as well as non-epithelial cell types, and in the apical membrane from human placenta. The human placental syncytiotrophoblast is the main barrier for maternofetal exchange. Annexin-A6 regulate the Cl − conductance of Maxi-chloride channel of apical membrane in human placenta [1312].
10.8.5.11 Annexin-A7
Annexin-A7170 participates in the control of Ca
release from internal stores by both inositol trisphosphate (IP3R) and ryanodine (RyR) receptors. Annexin-A7 associates with sorcin, an inhibitor of the coupling between of CaV1 and RyR2 channels in cardiomyocytes [1132]. Alternative splicing, which is tissue specific (brain, heart, and skeletal muscle) generates 2 isoforms.

Annexin-A7 promote the fusion of chromaffin granules to the plasma membrane in neuroendocrine cells of the adrenal medulla. Arachidonic acid and SNARE proteins promotes the fusion of membranes that come into contact via annexin-A7 [1304].
10.8.5.12 Annexin-A9
Annexin-A9,171 an atypical member of the annexin family, does not involve a membrane scaffold used by other annexins. It has 4 homologous calcium-binding sites in its tetrad core that also contains amino acid substitutions that ablate their function.
10.8.5.13 Annexin-A10
Annexin-A10172 has a restricted expression. It is synthesized in the adult liver, but not in multiple adult and fetal tissues.
10.8.5.14 Annexin-A11
Annexin-A11173 is a midbody protein involved in the cytokinesis stage of the cell division. It may intervene in the trafficking and insertion of vesicles during cytokinesis. It enters the nucleus at prophase, before localizing to the midbody. Annexin-A11 binds to S100a6 protein (calcyclin).
Annexin-A11 may be a target of S100a6 protein. It can be phosphorylated by mitogen-activated protein kinase; once phosphorylated by MAPK, it can still bind to phosphatidylserine-containing vesicles.
10.8.5.15 Annexin-A13
Annexin-A13174 can associate with membranes owing to an N-terminal myristoylation in a Ca
-independent manner. In particular, it is associated with the plasma membrane of proliferating endothelial cells. Alternative splicing generates different isoforms.

In epithelial cells, annexin-A13b splice variant links specifically to sphingolipid- and cholesterol-rich membrane domains of the trans-Golgi network [1304]. N-terminally myristoylated annexin-A13b is required for the budding of these domains, which are subsequently delivered to the apical plasma membrane.
10.8.5.16 Annexin Role in Ca + + Handling in Cardiac Cells
Heart cells contains annexin-A1, -A2, and -A4 to -A7. Endothelial cells express annexin-A1, -A2, -A5, and -A6; smooth muscle cells mainly annexin-A2 and -A6, and cardiomyocytes mostly annexin-A4 to -A7.
These annexin species modulate the cytosolic concentration of free Ca
ions with other Ca
handlers and Ca
sensors. Whereas Ca
-binding sorcin and AHNAK nucleoprotein, or desmoyokin, regulate CaV1.2 channels and calsequestrin, histidine-rich Ca
-binding protein and sorcin control sarco(endo)plasmic reticulum Ca
-release channels, and annexin-A2 and -A6 modulateNa + –Ca
exchanger activity [1315].







Annexin-A2
Annexin-A2 localizes to extracellular matrix and endothelial cells of coronary arteries. This cytoskeleton-binding protein below the plasma membrane can form heterotetramers with S100a10, thereby reducing Ca
requirement for phospholipid binding. Activated annexin-A2 operates as a plasmalemmal coreceptor for plasminogen and tissue-plasminogen activator [1315]. Annexin-A2 thus controls plasmin-mediated processes, hence acting as an anti-thrombolytic.175 In addition, annexin-A2 associates with HSP90a.

Annexin-A4
In fibroblasts, annexin-A4 impedes Ca
-dependent Cl − flux by precluding interaction between Ca
–calmodulin kinase-2 and ion channel and channel phosphorylation [1315]. In cardiomyocytes, cytosolic annexin-A4 level increases during heart failure.


Annexin-A5
Annexin-A5 abounds in human myocardium; it resides mainly in cardiomyocytes. In atriomyocytes, it may mediate release of atrial natriuretic peptide. Annexin-A5 is also present in vascular endothelial cells. It may have anticoagulant, anti-apoptotic, and anti-inflammatory effects [1315].
Annexin-A6
Annexin-A6 also abounds in the heart. In cardiomyocytes, it resides in the cytosol, sarcolemma, T tubules, and intercalated discs. It is also detected in various cell types, such as smooth muscle and endothelial cells. It is involved in secretion of atrial natriuretic peptide. Annexin-A6 relocalizes from the cytoplasm to plasma membrane in smooth muscle cells and cardiomyocytes during contraction. It has an indirect negative inotropic effect [1315].
Annexin-A7
Annexin-A7 is expressed in cardiomyocytes. It has a Ca
-dependent GTPase activity regulated by protein kinase-C. The annexin-A7–sorcin complex interacts with ryanodin (RyR2) and CaV1 channels and may act as a mediator of interchannel communication during excitation–contraction coupling.

10.9 Transcription Factors Involved in Stress Responses
Main participants of gene transcription include RNA polymerase, transcription factors, coactivators, and corepressors. Because DNA exists in a condensed state wrapped by histones that complex to form chromatin, chromatin remodelers with nucleosome disassembly associated with histone acetylases, deacetylases, kinases, and methylases must operate to free the access to RNA polymerase and transcription factors.176 Chromatin-restructuring complexes can be classified into 2 main categories: (1) ATP-dependent complexes, such as members of the SWI/SNF and ISWI (imitation SWI) remodeler families (Table 10.40), and (2) histone acetyltransferases and deacetylases.
Table 10.40.
Examples of ATP-dependent chromatin-remodeling complexes (Source: [1316]; ActL: actin-like protein; ARID: AT rich-interactive domain [SWI1-like]; CHD: chromodomain helicase DNA-binding protein; MBD: methyl-CpG (cytosine–phosphate–guanine)-binding domain protein; RBBP: nucleosome-remodeling retinoblastoma-binding protein; RSF: remodeling and spacing factor; SMARC: SWI/SNF-related, matrix associated, actin-dependent regulator of chromatin).
Type | Subunits |
---|---|
SWI2/SNF2 | ActL6a/b, ARID1a/b, SMARCa2/4, SMARCb1, SMARCc1/2 |
SMARCd1–SMARCd3, SMARCe1 | |
ISWI | RSF1, SMARCa5 |
CHD | CHD3/4, HDAC1/2, MBD3, RBBP4/7 |
The gene expression is controlled by transcription factors that depend on cell signaling, in addition to chromatin modifications, RNA splicing, and RNA control mechanisms. More than 2000 transcription factors are encoded in the human genome.
10.9.1 Nuclear Factor-κB Signaling Module
Nuclear factor κ light chain enhancer of activated B cells, or simply nuclear factor-κB (NFκB), was originally discovered as a transcription factor that regulates immunoglobulin gene expression. In fact, genes regulated by nuclear factor-κB in coordination with different signaling pathways control cell adhesion, survival, proliferation, apoptosis, and stress response, as well as tissue remodeling, inflammation, and innate and adaptive immunity. This ubiquitous, pleiotropic factor that constitute a family of dimeric transcription factors is thus involved in angiogenesis. Deregulated NFκB signaling is involved in malignant transformation, autoimmunity, chronic inflammation, metabolic disorders, and neurodegenerative diseases.
Factor NFκB corresponds to any dimeric transcriptional factor of the REL family. Five members are categorized into 2 structural groups (Table 10.41): group-1 NFκB factors, i.e., precursors NFκB1 (or P105NFκB monomer), which is processed into P50NFκB subunit by the 26S proteasome, and NFκB2 (or P100NFκB monomer), which produces P52NFκB subunit; and group-2 factors with RelA (P65), RelB, and Rel (or cRel). Subunits NFκB1 and NFκB2 actually give rise to P50NFκB and P52NFκB homodimers, respectively. Subunit P100NFκB selectively stabilizes RelB-containing dimers,177 although it can associate with and inhibits several NFκB family members [1317]. The prototypical nuclear factor-κB is the P50–RelA heterodimer.
Table 10.41.
The nuclear factor-κB (or REL) family of dimeric transcription factors and its structural categories. All family members contain an N-terminal Rel homology domain (RHD) that enables dimerization, nuclear localization, and DNA binding. Category-2 members possess a transcription activation domain (TAD) to activate gene expression. Both P50NFκB and P52NFκB are generated from precursors P105NFκB and P100NFκB, respectively, by proteasomal degradation of the C-terminal IκB-like domain. They then bind to a TAD-containing NFκB category-2 member to create a functional NFκB heterodimer.
Category | Types |
---|---|
1 | NFκB1 |
(P105NFκB precursor monomer processed to | |
generate P50NFκB dimer) | |
NFκB2 | |
(P100NFκB precursor monomer processed to | |
generate P52NFκB dimer) | |
2 | Rel |
RelA (P65NFκB or NFκB3) | |
RelB |
In resting cells, NFκB is inactive due to its sequestration in the cytosol by one of its inhibitors. Dimeric NFκB factors indeed complex with inhibitors of NFκB: typical IκBα, IκBβ, and IκBε, as well as atypical IκBδ (or IκBNS), IκBζ, and BCL3 (Tables 10.42 and 10.43). In addition, C-termini of P100NFκB and P105NFκB contain IκB-like ankyrin repeats that mimic the inhibition of IκB family members (with its 2 functional groups).
Table 10.42.
Functional categories of the IκB family of inhibitors of NFκB transcription factor (BCL3: B-cell lymphoma-3). Protein P105NFκB serves as a precursor for P50NFκB subunit (NFκB1) after limited proteolysis of P105NFκB monomer by the proteasome. On the other hand, K48-linked ubiquitination of P105NFκB by ubiquitin ligase SCF triggers its complete degradation by the 26S proteasome.
Category | Members |
---|---|
Typical IκB proteins | IκBα, IκBβ, IκBε |
Atypical IκB proteins | IκBδ, IκBζ, BCL3 |
NFκB precursors | P100NFκB, P105NFκB |
Table 10.43.
Inhibitors of NFκB that sequester the inactive transcription factor in the cytoplasm form a family (Source: [1317]). The IκB family includes: (1) typical IκB proteins (IκBα, IκBβ, and IκBε); (2) precursor proteins P100NFκB and P105NFκB; and (3) atypical IκB proteins (IκBδ, IκBζ, and B-cell lymphoma-3 (BCL3) that are expressed after cell stimulation, but generally not in unstimulated cells (inducible IκBs). Atypical IκB proteins reside in the nucleus rather than cytoplasm and have a more restricted cell expression pattern.
Protein | Function |
---|---|
IκBα | NFκB cytosolic sequestration, |
binding to RelA and Rel | |
IκBβ | NFκB cytosolic sequestration |
IκBε | NFκB cytosolic sequestration |
IκBδ | Homodimer P50–DNA binding (repression) |
IκBζ | Homodimer P50–DNA binding (transcription) |
P100NFκB | Release of P52NFκB (transcription) |
Formation of P52–RelB complex (repression) | |
Binding to RelB for P52NFκB activation | |
Binding to free NFκB dimers (repression) | |
P105NFκB | Release of P50NFκB (transcription) |
Binding to free NFκB dimers (repression) | |
BCL3 | Binding of P50–P50 and P52–P52 homodimers to DNA |
upon phosphorylation | |
(transcription) | |
P50NFκB cytosolic sequestration upon deubiquitination |
Typical IκB proteins sequester NFκB in the cytoplasm, whereas atypical IκB proteins act in the nucleus as NFκB coregulators. Ubiquitination regulates NFκB activity via proteasomal degradation of inhibitors upon activation of IκB kinase that phosphorylates IκB proteins.
Ubiquitination and subsequent degradation of inhibitors activate both canonical and non-canonical NFκB pathways. These 2 basic pathways are indeed triggered by the release of NFκB dimers from their tethered inhibitors. Activation of NFκB depends on its induction pattern. For example, NFκB regulation of Jun N-terminal kinase can have opposite effects according to the cell type.
In summary, the nuclear factor-κB signaling module is composed of: (1) homo- and heterodimeric transcription factors; (2) many regulatory subunits, NFκB-bound inhibitors of NFκB (IκB) that cause cytosolic sequestration in unstimulated cells (IκBα, IκBβ, IκBγ, IκBε, IκBζ, as well as nucleus-resident TLR-inducible IκBδ and BCL3), in addition to precursors P100NFκB and P105NFκB); and (3) inhibitor of NFκB kinase complexes such as the IKKα–IKKβ–IKKγ complex made of 3 IκB kinase subunits that comprise 2 catalytic components – IκB kinase subunits IKKα (IKK1) and IKKβ (IKK2) – and a regulatory protomer IKKγ178 In addition, the IκB kinase family contains another member, IKKε, that forms another IκB kinase complex [1318].
10.9.1.1 IκB Regulators
Proteins IκB are not simple inhibitors of NFκB activity, but pleiotropic NFκB cofactors and regulators of gene expression. The IκB family comprises 8 members that are classified into 3 functional groups [1319]: (1) precursor proteins (P100NFκB and P105NFκB); (2) typical IκB proteins (IκBα, IκBβ, and IκBε); and (3) atypical IκB proteins (IκBδ, IκBζ, and B-cell lymphoma protein BCL3).
Typical IκB Proteins
Typical IκB regulators IκBα and IκBβ have different preferences for NFκB dimers and experience distinct histories. The former associates with the Rel–P50 and RelA–P50 dimer, whereas the latter, in an hypophosphorylated form, enters into the nucleus and links to the Rel–RelA dimer [1319]. The linkage between IκBβ and Rel does not prevent DNA binding of Rel protein. Furthermore, the binding of the IκBβ–Rel–RelA complex to DNA resists to IκBα and may enhance the expression of genes. In addition, IκBβ is subjected to slow phosphorylation in the cytosol and degradation in a different manner than IκBα. Members of the typical IκB group can operate as both positive and negative NFκB regulators. Agent IκBβ specifically promotes TNFα transcription.
In cultured rat vascular smooth muscle cells, persistent activation of NFκB by IL1β relies on extracellular signal-regulated kinase. Interleukin-1β activates ERK then P90 ribosomal S6 kinase RSK1. The latter is a component of the RSK1–IκBβ–NFκB complex, whatever its phosphorylation status [1320]. Both active ERK2 and RSK1 phosphorylate IκBβ, but only active RSK1 phosphorylates IκBβ on Ser19 and Ser23, 2 sites that mediate the subsequent ubiquitination and degradation.
Atypical IκB Proteins
Members of the atypical IκB group act also as activators and inhibitors of gene expression. Atypical IκB proteins of the NFκB inhibitor family complex with inhibitory P50NFκB or P52NFκB homodimers [1317].
Iκ Bζ
The IκBζ protein complexes with P50 homodimers and RelA-containing dimers for transcription activation and repression, respectively.179 Agent IκBζ is not expressed constitutively, but rapidly produced after stimulations by lipopolysaccharide and interleukin-1 via specific NFκB signaling mediated by MyD88 adaptor. The IκBζ regulator is an activator or inhibitor of NFκB according to the context. It can associate with P50 homodimers bound to specific sites on the IL6 promoter to prime gene expression [1319].
IκBδ or IκBNS
Expression of IκBδ, or IκBNS, is associated with apoptosis of immature thymocytes. Agent IκBδ is rapidly expressed upon TCR-triggered thymocyte death that eliminates thymocytes with autoreactive T-cell receptors [1321]. Protein IκBδ activated by anti-inflammatory cytokines such as interleukin-10 can repress transcription by stabilizing P50 homodimers. Agent IκBδ inhibits the expression of NFκB target genes such as the interleukin-6 gene, but promotes that of a small set of genes [1319].
BCL3
B-cell lymphoma protein-3 of the IκB family possesses a typical transactivating domain (TAD) in its C-terminus. It thus yields a transactivating activity to P50 and P52 homodimers that lack a TAD domain, once it has experienced adequate post-translational modifications, such as phosphorylation and ubiquitination, especially following pro-inflammatory signaling.180
On the other hand, in the absence of proper post-translational modifications, BCL3 impedes NFκB-induced gene expression. Agent BCL3 stabilizes P50 homodimer occupancy of target gene promoters, as it prevents its ubiquitination and subsequent degradation, thereby precluding their replacement with active NFκB dimers. Upon stimulation by anti-inflammatory cytokines, ubiquitinated, non-phosphorylated BCL3 may also stabilize P50 and P52 homodimers, hence functioning as an inhibitor. On the other hand, cylindromatosis deubiquinase (Cyld) causes cytosolic sequestration of the BCL3–(P52)2 and BCL3–(P50)2 trimers.
Activation of B and T lymphocytes by a large number of extracellular stimuli, such as cytokines IL1 and TNFα, bacterial lipopolysaccharides, and virus, causes the phosphorylation of 2 serines near the N-terminus of IκBα (Ser32 and Ser36). This phosphorylation leads to the ubiquitination of 2 lysines on IκBα (Lys21 and Lys22) by the ubiquitin ligase complex SKP1–cullin–F-box β-transducin repeat-containing protein (SCFβTRCP) and its degradation by the 26S proteasome.
10.9.1.2 IκB Kinase
Both IKKα and IKKβ isozymes phosphorylate IκB proteins, thereby releasing NFκB dimers (Table 10.44). Repressor IKKα limits NFκB stimulation. The regulatory IKKγ subunit is required for NFκB activation.
Table 10.44.
Family of IκB kinases.
Type | Effect |
---|---|
IKKα | Phosphorylation of IκB (repression) |
IKKβ | Phosphorylation of IκB |
IKKγ | Regulatory subunit |
(NFκB essential modulator NEMo) | |
IKKε | NFκB stimulation by T-cell receptor |
In addition, IKKα and IKKβ phosphorylate NFκB proteins, in particular RelA to regulate the recruitment of transcriptional coactivators and corepressors [1317]. Phosphorylated cAMP-responsive element-binding (CREB) protein-binding protein (CBP) binds preferentially to RelA w.r.t. other transcription factors such as P53 (P53–NFκB cross-regulation).
Activation of the IKK complex in response to 12Otetradecanoylphorbol 13-acetate (TPA or phorbol 12-myristate 13-acetate [PMA]) is mediated by protein kinase-Cα [1322].
IKKα
Enzyme IKKα also targets corepressor complex silencing mediator of retinoic acid and thyroid hormone receptor (SMRT) that links repressive NFκB complexes such as P50 homodimers and histone deacetylase-3 to remove HDAC3 and replace P50 homodimers with NFκB activators such as the P50–RelA complex.
In the nucleus, IKKα can phosphorylate histone-3 for greater κB-site accessibility [1317]. Furthermore, IKKα can activate TNFSF11 to inhibit the transcription of serpin-B5, which is regulated by P53, without intervention of NFκB dimers. Therefore, IKKα can associate with serpin-B5 gene promoter and then prevent gene expression independently of NFκB.
The IKKα kinase is not required for cytokine-dependent activation of NFκB, but does intervene in keratinocyte differentiation [1318].
IKKβ
Kinase IKKβ is required for activation of NFκB by tumor-necrosis factor-α (TNFSF1), but not interleukin-1 in embryonic fibroblasts.
IKKε
Kinase IKKε is involved in NFκB stimulation by T-cell receptor, but neither by TNFα nor IL1 cytokines.
Trimeric inhibitor of nuclear factor-κB kinase intervenes not only in transcription by NFκB, but also in signaling mediated by MAPK modules, Toll-like and T- and B-cell receptors, insulin, and adipocytokines. Both IKKε and TRAF family member-associated NFκB activator (TANK)-binding Ser/Thr protein kinase TBK1 transmit signals that emanate from pattern recognition receptors leading to the phosphorylation of IRF3 transcription factor.
Kinase IKKε also acts in cell apoptosis and substance transport [1323]. Endocytosis as well as cytokinesis and cell migration rely on the spatiotemporal regulation of transmembrane transfer events (localized recruitment and activation of molecules and coordination of their activities). Kinase IKKε phosphorylates Rab11 effectors. Localized activation of IKKε can regulate the delivery of Rab11 + vesicles derived from recycling endosomes.181
10.9.1.3 Types of NFκB Signalings
Two NFκB signaling pathways exist: classical or canonical and alternative or non-canonical (Tables 10.45 and 10.46). Canonical and non-canonical NFκB signaling are responsible for the release of NFκB dimers from their inhibitors.
Table 10.45.
Features of the canonical and non-canonical NFκB pathways (Part 1; Sources: [1324, 1325]; IKK: IκB kinase; MAP3K: mitogen-activated protein kinase kinase kinase). Upon canonical IKK activation, IκBα, IκBβ, and IκBε that sequester NFκB components into the cytoplasm are phosphorylated on specific N-terminal residues that are docking sites for the SCFβTRCP ubiquitin ligase complex, hence labeling for proteasomal degradation. Four transcriptional activators (RelA–RelA, P50–RelA, Rel–Rel, P50–Rel) are then formed. Factor NFκB provokes production of IκBα and IκBε, thereby yielding a negative feedback. The non-canonical pathway depends on the protein–ubiquitin ligase complex composed of inhibitors of apoptosis (IAP1 and IAP2) as well as tumor-necrosis factor receptor (TNFR)-associated factors (TRAF2 and TRAF3). Disruption of the TRAF2–TRAF3–IAP complex triggers the non-canonical NFκB signaling. Persistent degradation of this complex enables a sustained signaling. Kinase MAP3K14 phosphorylates P100NFκB (Ser866 and Ser870), thus activating IKKα. Activated IKKα-containing IKK complex further phosphorylates P100NFκB (Ser99, Ser108, Ser115, Ser123, and Ser872) that is then recognized by the SCFβTRCP ubiquitin ligase complex for partial degradation by the 26S proteosome, hence allowing nuclear translocation of the major P52–RelB and minor P52–RelA dimers that act in the late sustained phase. Degradation of IκBδ enables release of P50–RelB and P50–RelA dimers in the early phase.
Feature | Canonical axis | Non-canonical axis |
---|---|---|
Occurrence | Rapid | Slow |
Independence of | Dependence of | |
protein synthesis | protein synthesis | |
Duration | Transient | Sustained |
Regulated | Inflammation, | Lymphoid organogenesis, |
functions | Immunity | B-cell survival and maturation, |
dendritic cell activation, | ||
bone metabolism | ||
Components | MAP3K7 | MAP3K14 |
(kinases) | IKKβ/γ | IKKα |
Targeted | IκBα | P100NFκB |
inhibitor | (predominantly) | |
Transcription | P50–RelA | P52–RelB |
effector | (predominantly) | (prototypical heterodimer) |
Table 10.46.
Features of the canonical and non-canonical NFκB pathways (Part 2; Sources: [1324, 1325]; DAMP: danger-associated molecular pattern; NLRP: NLR family, pyrin domain-containing protein; PAMP: pathogen-associated molecular pattern; TNAP: TRAF- and NIK-associated protein; TNFRSF: tumor-necrosis factor (TNF) receptor superfamily member; ZFP: zinc finger protein).
Feature | Canonical axis | Non-canonical axis |
---|---|---|
Activating | Cytokines, | Agonists of some TNFRSFs |
substances | PAMPs, DAMPs | |
Triggering | Various | Subset of TNFRSFs |
receptors | (TNFRSF1b/3/5/7/8, | |
TNFRSF11a/12a/13C) | ||
Ubiquitinases | SCFβTRCP | SCFβTRCP |
(IκB degradation) | (P100 cleavage) | |
TRAF2/5 (TNFR) | TRAF2/3, IAP1/2 | |
IAP1–UbE2d [RIPK1] | ||
LUbAC [IKKγ] | ||
TRAF6–UbC13 (TLR/IL1R) | ||
[IRAK1] | ||
Regulators | NLRP12, TNAP | |
(MAP3K14 inhibitors) | ||
ZFP91 | ||
(MAP3K14 activator) | ||
Feedback | NFκB ⊕ →IκBα/ε | IKKα → MAP3K14 |
Canonical NFκB Pathway
The canonical NFκB pathway is related to P50–RelA and P50–Rel heterodimers. In resting cells, inhibitory IκB binds to NFκB dimers, thereby sequestering these dimers in the cytoplasm. Subunit IKKβ is necessary and sufficient to phosphorylate IκBαin cooperation with IKKγ. The IκB kinase complex phosphorylates IκB that is then polyubiquitinated by SCF ubiquitin ligase for proteasomal degradation, thereby activating NFκB factor. Multiple negative feedbacks control the canonical NFκB signaling.
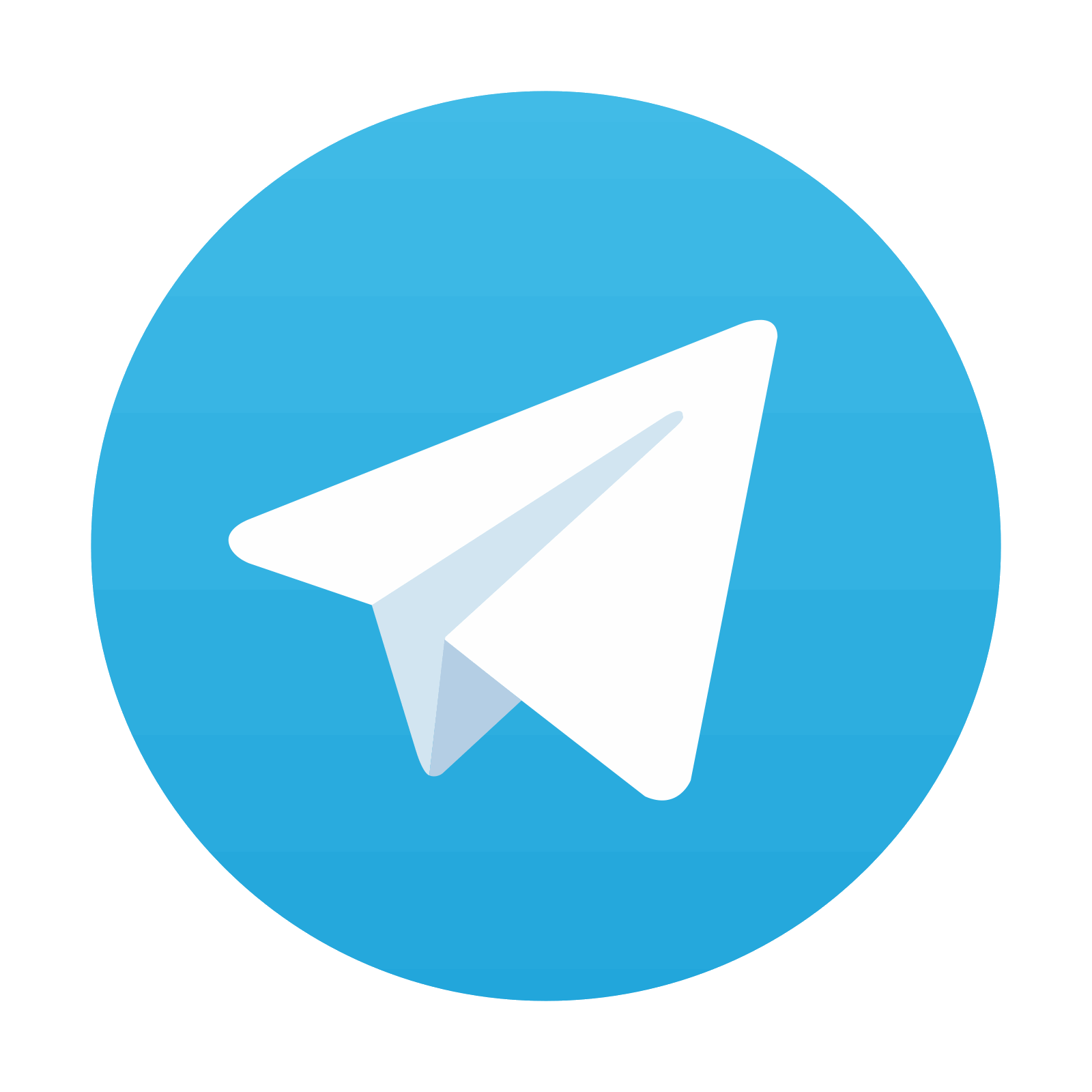
Stay updated, free articles. Join our Telegram channel
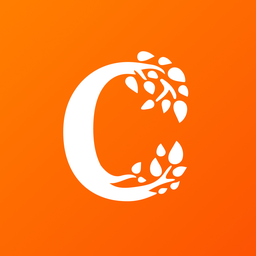
Full access? Get Clinical Tree
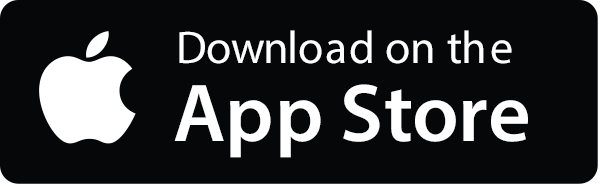
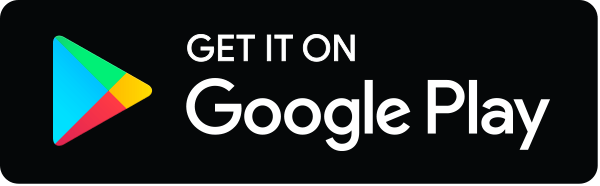
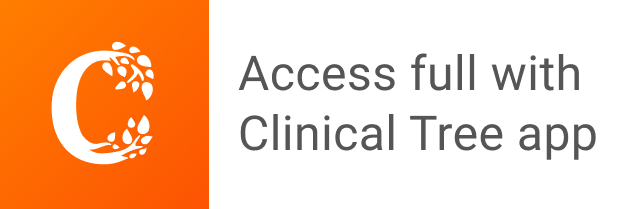