CHAPTER 12 The human skeleton, consisting of the craniofacial bones, the axial skeleton, and the appendicular skeleton, arises from cells of three distinct embryonic lineages. Only the development of the axial and appendicular skeleton will be discussed here. Development of the axial skeleton can be divided into three stages that begin at week 4 after gestation.173 During the “pre-cartilaginous stage” (weeks 4–6), cells from the paraxial mesoderm condense to first form somites, or bilateral progenitor bodies, and then sclerotomes which surround the notochord and become precursors for the vertebral bodies and most of the skull. Sclerotomes also differentiate into chondrocytes, which migrate around the notochord to form the neural arches, ribs, and transverse processes. During the “cartilaginous stage” (week 7), the embryonic structures, or anlagen, are replaced by cartilage produced by the chondrocytes. In the final “bony stage” (week 7 to year 25), three primary ossification centers from each cartilaginous vertebra play different roles in forming the bony spine. One ventral ossification center becomes the vertebral body, while two dorsal ones fuse together to form the two vertebral arches by the third to fifth year of life. Secondary ossification centers ensure that vertebral development continues well into puberty. The appendicular skeleton is formed by cells that originate from the lateral plate mesoderm.129 The shaping of limbs during development depends on growth factors from specialized cells in the environment. Fibroblast growth factor (FGF) directs specialized epithelial cells in the apical ectodermal ridge58 at the tips of limb buds to undergo proximodistal outgrowth, while anteroposterior growth is directed by the sonic hedgehog (SHH) chemokine. The limb bones are formed in an orderly fashion with the humerus/femur first taking shape. Phalangeal anlagen are formed last, and segmentation and formation of joints are produced by apoptotic cell death. During limb development, cells from the somite migrate to the embryonic appendages and develop into nerves, blood vessels, and muscles. The terms “osteoporosis” and “osteopenia” refer to the loss of bone mass and strength, resulting in an increased risk of fractures. The World Health Organization (WHO) defines the two terms using a T-score, which describes the bone mass of an individual relative to a comparative population of age 20.170 A T-score<–1.0 means having a bone mass less than 1.0 standard deviations44 below the mean for young healthy adults of the same gender, defined by the NHANES reference database on Caucasians 20–29 years old.102 A T-score<–2.5 represents having a bone mass of 2.5 standard deviations below the benchmark population. The reference site of bone mass density (BMD) measured by dual-energy x-ray absorptiometry (DEXA) scan is the femoral neck, but other central sites (lumbar spine, total hip) may be used for clinical diagnosis. Osteoporosis is separated into two broad categories. Primary or idiopathic osteoporosis typically refers to age-related changes in bone remodeling that are exaggerated and pathologic.63 Two types of primary osteoporosis are post-menopausal osteoporosis and senile osteoporosis. Post-menopausal osteoporosis occurs in women between 51 and 75 years old, and is linked to estrogen deficiency accompanying menopause. Senile osteoporosis usually occurs in those older than 60 years old, affects both men and women, and has a different pathophysiology of reduced bone turnover due to reduction in the number of osteoblasts. Secondary osteoporosis describes bony degenerative changes not directly related to age. Some common causes include medications such as diuretics, endocrine disorders, and congenital abnormalities.77 Up to one-third of those with idiopathic osteoporosis also have a co-existing condition contributing to bone loss, which exacerbates the expected decrease in BMD that accompanies age. The worldwide prevalence of osteoporosis is estimated to be over 200 million.34 In the United States alone, at least 8 million women and 2 million men have osteoporosis. An additional 18 million people have osteopenia, which put them at increased risk of continuing to lose BMD.101 The US Preventive Services Task Force (USPSTF) estimates that half of all post-menopausal women are expected to have at least one osteoporosis-related fracture in their lifetime, of which 25% will suffer from a vertebral deformity and 15% will experience a hip fracture.101 This tremendous prevalence contributes to at least 1.5 million fractures each year, with an estimated annual cost of $17 billion.47 As the population continues to age, the rate and burden of osteoporosis and osteopenia will continue to increase. Osteoporosis is essentially a consequence of imbalance between bone resorption and formation during bone remodeling.63, 138 Osteoclasts, which are macrophage-like cells found in the lacunae, initiate the resorption process by initially acidifying the extracellular compartment causing demineralization. The process continues with degradation of the organic matrix by cysteine proteases. After fulfilling their function, osteoclasts undergo apoptosis and are subsequently replaced by osteoblasts, fibroblast-like cells that replete the resorbed bone by first depositing osteoid (unmineralized bone matrix), which then undergo mineralization to maintain bone density and strength. Bone density is well maintained during young adulthood, when bone resorption is slightly less than bone growth. However, after the ages of 30–45, resorption exceeds formation and ultimately leads to osteopenia/osteoporosis and increased fracture risk. The degree of bone loss depends on multiple factors including age, gender, medical history, lifestyle, and body sites, all of which determine signaling regulators controlling this dynamic process.101, 111 In recent years, genome-wide association studies have identified specific loci and genes associated with BMD, which has historically been observed to be highly heritable.12, 44, 145 The most common fractures sites from osteoporosis are the vertebrae, the hips, and the wrist, with incidences of 700,000, 300,000, and 250,000 cases per year, respectively.101 Vertebral compression fractures can cause kyphosis, height loss, and other changes in body habitus that may result in decreased ambulatory abilities and further trigger pulmonary and gastrointestinal dysfunctions.63 Hip fractures lead to permanently diminished mobility in 50% of all patients, dramatically increase the incidence of deep vein thrombosis and pulmonary embolism, and are associated with a mortality rate of 5–20% in the post-surgical year. Pain, the most common symptom of osteoporosis-related fractures, reduces ability to work, limits social activities, and reduces the general quality of life.33 Elderly patients’ abilities to carry out activities of daily living are greatly diminished even after recovery from fractures, while their mortality risk is increased by 5–10 years with each low-impact fracture.20 Preservation of BMD is crucial in the prevention of pathological fractures. In a study of post-menopausal women older than 50 years, fractures were associated with approximately 4- and 1.8-fold increase with osteoporosis and osteopenia, respectively.170 More importantly, almost half of the population had undetected low BMD. The USPSTF recommends that all women over 65 be routinely screened.47 Osteoporosis is the endresult of an interplay between non-modifiable (i.e. age, gender, ethnicity, genetics) and modifiable factors. Current recommendations are to consume 1,000 mg of calcium and 600 units of vitamin D daily in men older than 50, 1,200 mg of calcium and 600 units of vitamin D daily in women older than 50, and 1,200 mg of calcium and 800 units of vitamin D daily in those older than 70.2 Additionally, increase in protein intake and decreases in alcohol and caffeine intake are associated with decreasing fracture risks.62, 85, 119 Mediations such as steroids, heparin, certain anti-epileptics and many others may exacerbate bone loss, so preventive measures are especially important for these patients.117 Regular high-impact exercise and smoking cessation have been correlated with higher BMD.35, 68 Regulation of bone density and strength was first postulated in 1892 by Julius Wolff, whose “law” attributed bone growth to proportional mechanical loading.196 In vitro and in vivo studies of bone remodeling, partly encouraged by the need to elucidate measures to prevent severe osteoporosis in astronauts from prolonged space travel,1 led to the recognition of fluid flow as another important regulator of the dynamic process.70, 142 Rather than two distinct forces, mechanical stress exerts its effect through altering fluid flow to regulate remodeling and growth.130 Bone remodeling is a five-step process based on the intricate dynamics of three cell types.138 The activation phase begins with the detection of remodeling signals by osteocytes, which compose more than 95% of all skeletal bone cells and have long dendrites that extend to bone marrow and surface.23 Signals take on many forms, from mechanical stress causing structural damage to hormones (e.g., PTH or estrogen) induced by homeostatic changes. In the resorption phase, osteocytes and their precursor osteoblasts respond to molecular signals (e.g., parathyroid hormone) by producing cytokines, notably monocyte chemoattractant protein-1 (MCP-1, chemoattractant for osteoclast precursors), colony stimulating factor 1 (CSF-1) and receptor activator of nuclear factor κB ligand (RANKL, which works to promote survival, proliferation, and differentiation of osteoclast precursors into osteoclasts), and reducing the production of OPG (which inhibits osteoclast proliferation).75, 99, 107 These molecular changes increase the presence of both precursor and mature osteoclasts, which then attach to the leading front of a basic multicellular unit (BMU), a temporary structure created from the aforementioned cell types during bone remodeling. The reversal phase thenensues with the digestion of demineralized collagen matrix by “reversal cells,” likely of osteoblastic lineage. Transition from bone resorption to formation within the BMU is orchestrated by coupling signals. The formation phase is defined by the production mainly of collagen type I, the main organic bone component, and non-collagenous proteins such as proteoglycans, by activated osteoblasts.114, 124 These molecules conjugate together to form osteoid, which is the foundation for subsequent hydroxylapatite deposition of an unknown mechanistic nature. In the final step, the termination/quiescence phase, mature osteoblasts cease activity, undergo apoptosis, or differentiate into osteocytes after being surrounded in mineralized bone matrix.138 Alteration of intramedullary fluid flow by mechanical loading to assist diffusion in delivering nutrients to bone cells was first proposed by Piekarski& Munro in 1977, from the observations that diffusion was a slow process and bones undergoing cyclic loading grew thicker and healthier while immobilization resulted in atrophy.81, 134 Subsequent studies attempted to decipher the mechanism of nutrient delivery in relation to fluid flow, focusing on aspects of bone porosity, protein penetration, and response to fluid shear stress.44, 156, 157 In vitro experiments demonstrated the ability of bone cells to detect flow stimulation and respond by moderating gene expression, similar to endothelial cells.91, 140–142 Flow-induced mechanotransduction depends both on the cell type (i.e., osteocytes, osteoblasts, osteoclasts) and the type of flow dynamics (i.e., steady, oscillating, or pulsing).78 The characteristics of strain experienced by bones depend on the nature of physical activity. On minor exertion such as standing, bones experience continuous extremely low-magnitude, high-frequency stress as a product of constant muscle contractions to maintain posture and encourage blood and lymphatic flow.74 Under heavy stress, bones can withstand approximately 0.7% deformation before irreversible damage. During these times, they will experience peak loading with low frequencies.4, 48 The importance of mechanical loading in retaining skeletal integrity is demonstrated by rapid bone loss experienced even from the lack of static or holding posture, such as chronic bed rest or cast immobilization.86, 96 Bone loss is proportional to muscle atrophy, which is why strength training is encouraged as a means of preventing osteoporosis.74 Load stresses experienced by bone cells are amplified by the extensive network of canaliculi and interstitial fluid surrounding them. Movement of intraosseous fluid from positional changes and muscular contractions generates dynamic fluid movement to induce shear stress. Oscillating fluid flow appears to generate more biochemical responsiveness in bone cells compared to constant flow, suggesting that dynamic mechanical signals are required for bone remodeling and adaptation.30, 36, 189, 201 Interestingly, chronic unloading can reduce the ability of blood vessels to increase blood flow by inducing increased vascular resistance.175 This leads to reduced nutrient delivery, possibly by limiting canalicular flow velocity, the existence of which has been shown to enhance the transport of sodium compared to diffusion alone.137 Bones are made up of two different layers, an outer layer made of compact bone (the cortex) and an inner spongy bone layer that encloses a network of trabeculae in a honeycomb-like structure. Long bones all have a similar structure: a long shaft or diaphysis, and round ends which typically have larger diameters than the shaft and are called epiphyses. Compact bone makes up the thick outer layer of the diaphysis, and at the center is the medullary cavity, which contains either yellow (fatty) or red (hemopoietic) bone marrow. The interiors of the epiphyses and the ends of the diaphysesare composed of trabecular bone. The outer layer of the epiphyses is made up of a layer of compact bone that is much thinner than the layer found around the diaphysis. The region in between the diaphysis (shaft) and epiphyses (ends) of the bone is the metaphysis and is separated from the epiphysis by the physisin growing bone, which gradually disappears as the bone matures. The outer surface of the bone is lined with a thin membrane called the periosteum.28, 112 The vascular anatomy of a typical long bone can be divided into three separate systems: afferent vessels, the microvascular network, and efferent vessels. Blood supply to the bone comes from the afferent vessels, which include the nutrient artery, epiphyseal arteries, metaphyseal arteries, and periosteal arteries.28, 118 Ion exchange between the blood and bone marrow occurs only in the microvascular network, which is composed of capillaries and sinusoids. The venous system includes the large central sinuses, as well as the epiphyseal, metaphyseal, periosteal, and nutrient veins.27, 93 The nutrient artery is one of the main blood supplies to long bone; it typically enters the bone halfway along the diaphysis at a shallow angle.37, 118 Once it reaches the medullary cavity, it splits into central ascending and descending longitudinal arteries that run parallel to the shaft, along the central longitudinal axis of the marrow, and alongside the central venous sinus. Small branches come off the longitudinal artery and run radially outward into both the marrow and into the encasing compact bone where they eventually branch off into small vessels that supply blood to the Haversian canals.103, 190 Nutrient veins accompany the nutrient artery through the central sinus and into the smaller radial branches into the cortex.127 Compact bone is made up of many osteons, which are long cylindrical units that run parallel to the bone shaft. At the center of the osteon is the Haversian canal, which contains both arterial and venous blood vessels as well as nerve fibers. Capillaries have also been observed inside the Haversian canals, representing the exchange vessels within the cortical bone. Oriented roughly perpendicular to the Haversian canals are Volkmann’s canals which connect the Haversian canals and the medullary cavity to the nerves and blood vessels outside of the bone.112 Arterial flow through healthy bone is centrifugal, coming from the nutrient arteries in the medullary cavity and going outward through the cortex.27, 118 In addition to the main nutrient artery, there are numerous smaller epiphyseal and metaphyseal arteries supplying blood to the ends of the bone.28, 37, 93 On the periosteum, a network of small periosteal vessels surrounds the bone. The extent to which the periosteal vessels supply blood to the bone is not clear; some have argued a “dual supply” theory where periosteal flow complements the centrifugal blood flow from the medullary cavity, while others have argued against this. While the full extent of the function of the periosteal vessels is not fully understood, they appear to supply blood at least to the outer surface of the bone.127, 187 Some evidence suggests that in certain conditions such as peripheral artery disease, where arterial supply to the medulla may be impaired, the periosteal arterial supply may be enhanced. The increased periosteal blood supply acts as a compensatory mechanism and may effectively reverse the typical centrifugal blood flow direction.27 The venous or vascular sinuses are thin vessels that connect to the central venous sinus, which runs parallel to the longitudinal artery in the marrow. The central venous sinus collects the venous flow from the many smaller veins in the diaphyseal marrow.93 It connects directly with the nutrient vein as well as numerous metaphyseal and epiphyseal veins, and it appears to also be connected to the periosteal veins.13 It is thought that the cortical capillaries, on the other hand, connect with the periosteal capillaries, which drain to intramuscular veins.28 While this classification of the vascular system in bone is helpful in understanding the overlying structure, it is important to realize that in reality the vascular network has numerous anastomoses that create interconnections between various branches of the vascular tree.28, 93 Additionally, there is still some debate as to the relative importance of the different vascular branches as well as to their functional anatomy.118 Bone blood circulation is under the control of several different mechanisms including neural, humoral, and metabolic processes.167, 168 Bone is highly innervated with sympathetic fibers, and while nerve fibers are found throughout, they are denser in the periosteum, metaphyses, and epiphyses than in cortical bone.67, 118, 162 Electrical stimulation of bone nerve fibers has been shown to result in vasoconstriction and reduced blood flow in different mammals such as cats, dogs,21, 22, 41, 42 and rabbits167 as measured by decreased outflow from the bone or a decrease in intramedullary pressure. A drop in the intramedullary pressure is known to correlate with a decrease in blood flow through the bone, as the pressure difference that drives the centrifugal flow is decreased.166 Additionally, increased bone flow has been observed after complete sciatic nerve section in rabbits.167 Bone blood vessels have been shown to respond to a number of vasoactive substances. Early studies have shown a fall in blood flow and intramedullary pressure in response to injection of adrenaline.13, 161, 166 More recent studies have demonstrated vasodilation in response to nitroglycerin and nitric oxide release, and vasoconstriction in response to endothelin.26, 115 Overall, it appears that bone is more sensitive to vasoconstrictors than to vasodilators.26, 46, 118 There are several metabolic factors such as blood pH, oxygen concentration, carbon dioxide concentration, and acid metabolites that have been shown to affect bone blood flow. In general, decreasing the oxygen concentration or increasing the carbon dioxide content results in increased arterial flow. Reactive hyperemic responses have also been observed in bone, and these remained even after cutting the nerves, suggesting a strong metabolic control mechanism that does not depend on neural innervation.28, 166 The myogenic effect, first reported by Bayliss in 1902, describes the natural tendency of blood vessels to vasodilate in response to a decrease in transmural pressure and to vasoconstrict in response to an increase in transmural pressure.16 Although the exact mechanism is not yet fully understood,38, 69, 159 it has been shown to be an important mechanism regulating blood flow in skin, muscle, and many other organs.24, 25, 79 While the myogenic effect may be enhanced during sympathetic stimulation, it is a separate mechanism that can be seen even when all innervation has been cut.22, 41, 42, 166 Since the blood vessels inside the bone are encased in the stiff bone structure, it is unclear exactly how a transmural pressure difference that activates the myogenic effect might be transmitted to these vessels. Yet some recent data indicate that the myogenic effect may indeed also occur in bone blood vessels, though the mechanism by which it is activated has only been hypothesized.116 Prolonged weightlessness experienced by astronauts during space missions leads to rapid and severe osteoporosis of normally weight-bearing bones, of which many are not completely reversible.71, 72 To develop countermeasures, both ground-based and space-flight animal models have been utilized to better understand bone adaptation. These in vivo studies elucidated the effect of perfusion and interstitial fluid flow on bone remodeling. One of the most widely used rodent models to simulate microgravity is hindlimb unloading.120, 121 Developed in the mid-1970s by the National Aeronautics and Space Agency (NASA) Ames Research Center, the model has been widely accepted as a valid simulation of microgravity. Rodents are suspended by attaching their tails to a pulley, allowing them mobility, grooming, and feeding using their forelimbs. This model offers a variety of advantages. First, a head-down position generates cephalic fluid shift, which occurs in absence of gravity.122 Second, forearms bear normal load and provide internal control.64 Third, hindlimb suspension enables further manipulation.120 Fourth, the model does not induce extra stress on the animals, as determined by corticosteroid levels and weight gain.60, 199 Unloading of the hindlimb leads to diminishing skeletal mass and bone mineral content as expected.56, 61, 185 Particularly, bone radius is diminished in both the metaphysis and the diaphysis; cortical and cancellous bones are similarly affected.56 Bone length remains unaffected, suggesting that factors other than load determine longitudinal growth.110, 174, 181, 182 The observed changes in bone structure may be mediated by decreases in osteoblast proliferation, maturation, and activity.19, 109, 206 Influences on osteoclast number and bone resorption are not consistent, and increases may reflect the amount of stress felt by the animals rather than the lack of load stimulus.61, 108, 110, 184, 186, 199 Decrements in bone mass increase the risk of fracture or bending, even at lower loads and in as little as two weeks.3, 113, 165, 182 Finally, unloading for 14 days followed by equal or longer duration of reloading may not adequately reverse the remodeling, despite rapid activating of bone formation rate at the initiation of reloading.163, 185 Studies assessing the significance of fluid dynamics on bone remodeling independent of weight bearing have demonstrated the ability to alter bone remodeling through perfusion alone. In early experiments, it was discovered that fractures healed at an accelerated rate distal to a venous tourniquet, presumably due to increased interstitial fluid flow.70, 92 Unloading of the hindlimbs immediately decreased mean arterial flow, which continued to diminish until a steady state of ~60% control value was reached in five days. When unloading was stopped, blood flow returned to pre-suspension levels, suggesting a possible role of blood flow in the etiology of both muscle atrophy and bone loss.148 Ligation of one femoral vein in hindlimb unloaded animals, while using the other femur as control, resulted in increased bone mineral content, length, and distal femur width. Vein ligation significantly elevated interstitial fluid flow (IFF) in the affected limb compared to the control.18 The difference in bone structure was attributed to (IFF), which is the movement of intramedullary fluid surrounding osteocytes, osteoclasts, and osteoblasts. Further evidence demonstrating the effect of perfusion and IFF on bone remodeling comes from following changes in structure and composition in bones that are normally partial or non-weight-bearing. Tailsuspension induces bodily fluid redistribution, resulting in cephalad fluid shift from the lower extremities to the head and upper body.64, 160, 193, 194 The mass of lower extremities substantially diminished as expected. Correspondingly, increases in the mass of bones in the upper bodies were observed. Hindlimb unloading alters only fluid flow without the addition of external pressure.31, 147 Since cranial bones are normally non-loaded while the humerus are partial-loaded compared to bones of the lower body, the observed increasesare solely attributable to the head-ward fluid shift and increased perfusion. Bed-rest studies in humans are designed to simulate physiological changes that accompany prolonged stay in microgravity.133 Many studies tilt the head of the bed downward by 3–6° to better simulate space travel. Maintaining a supine position for an extended time period induces similar physiological effects as seen in astronauts returning from missions. Specific to the effects on bone demineralization, extended immobilization and decreased lower-body fluid pressure induced muscle atrophy especially in the lower extremities, increased fatigability, increased calcium excretion, and mineral loss of the weight-bearing bones.88, 136, 146, 171 Comparison of the results from previous bed-rest studies and actual spaceflight demonstrates that the simulated microgravity model actually underestimates the magnitude of bone loss from spaceflight.14, 96, 97, 172
OSTEOPOROSIS, CIRCULATION,
AND FLUID DYNAMICS
12.1 Bone Development
12.2 Osteoporosis
12.2.1 Background and definitions
12.2.2 Epidemiology
12.2.3 Pathophysiology
Non-Modifiable
Potentially Modifiable
History of fracture as adult
Current cigarette smoking
History of fracture in first-degree relative
Alcoholism
Female gender
Impaired eyesight despite adequate correction
Advanced age
Recurrent falls
White ethnicity
Inadequate physical activity
Dementia
Poor health/frailty
Low body weight (<58 kg, or <127 lbs)
Estrogen deficiency
Early menopause (<45 years) or bilateral ovariectomy
Low calcium intake
12.2.4 Clinical consequences
12.2.5 Contributing and preventative factors
12.3 Bone Fluid Flow and Cellular Physiology
12.3.1 Bone remodeling
12.3.2 Mechanical loading and fluid flow
12.4 Bone-Blood Circulation
12.4.1 Vascular anatomy of the long bone
12.4.2 Mechanisms regulating bone circulation
12.5 Animal Studies of Bone Loss
12.5.1 Ground-based experiments
12.6 Clinical and Regenerative Medicine
12.6.1 Bed-rest studies
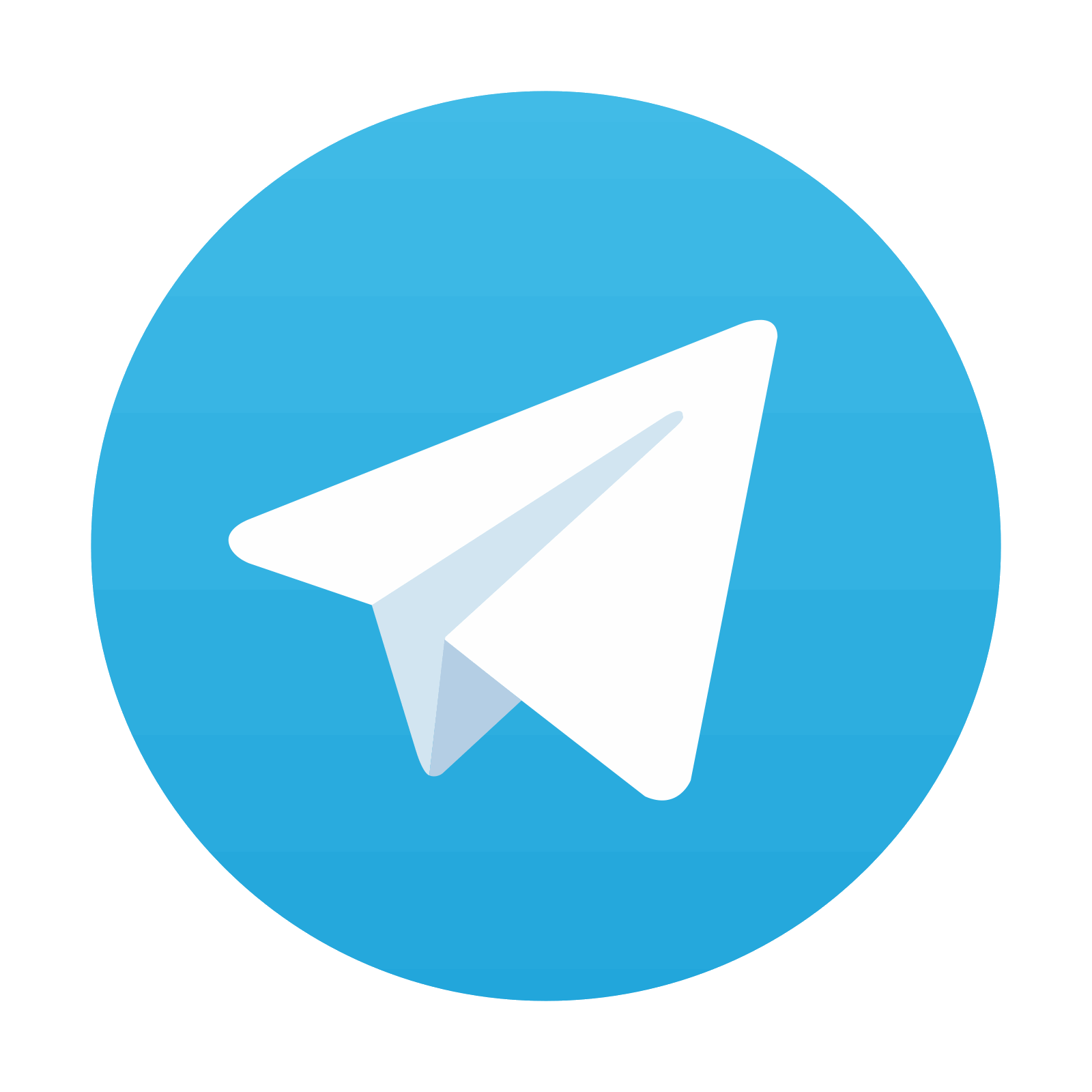
Stay updated, free articles. Join our Telegram channel
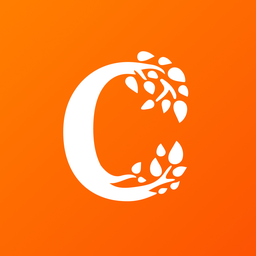
Full access? Get Clinical Tree
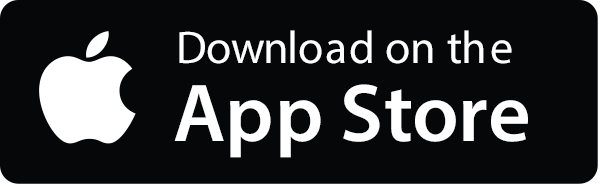
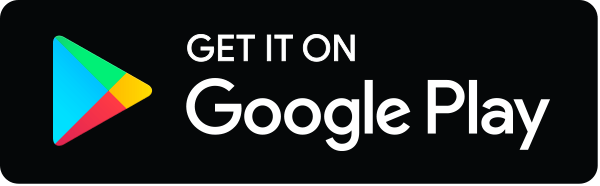