, Jagat Narula2, Yuliya Vengrenyuk3 and Samin Sharma4
(1)
Director, Cardiac Catheterization Laboratory, Director, Structural Heart Intervention Program, Director, Interventional Cardiology Fellowship Program, Zena and Michael A. Wiener Professor of Medicine, Icahn School of Medicine at Mount Sinai, Mount Sinai Hospital, New York, New York, USA
(2)
Director, Intravascular Imaging Core Laboratory, Instructor, Department of Medicine, Icahn School of Medicine at Mount Sinai, Mount Sinai Hospital, New York, New York, USA
(3)
Philip J. and Harriet L. Goodhart Chair in Cardiology, Chief of Cardiology, Mount Sinai St. Luke’s Hospital, Professor of Medicine and Radiology, Associate Dean, Arnhold Institute for Global Health, Icahn School of Medicine at Mount Sinai, Mount Sinai Hospital, New York, New York, USA
(4)
Director, Clinical and Interventional Cardiology, President, Mount Sinai Heart Network, Dean, International Clinical Affiliations, Anandi Lal Sharma Professor of Medicine, Icahn School of Medicine at Mount Sinai, Mount Sinai Hospital, New York, New York, USA
Keywords
Near-infrared lightOcclusive balloonPenetration depthCoronary plaqueFibrousLipid-richCalcificationMacrophage accumulationWhite and red thrombusStent malappositionEdge dissectionStent strut coverageIn-stent restenosisImaging artifacts1.1 Optical Coherence Tomography Principles
Optical coherence tomography (OCT) uses near-infrared light to generate high-resolution images of coronary arteries in vivo [1, 2] and can be considered an optical analog of intravascular ultrasound, which uses light instead of sound. The near-infrared light with a wavelength of about 1.3 μm is invisible to the human eye. OCT uses low-coherence interferometry by measuring the echo time delay and intensity of the light reflected from internal structures in tissue to generate cross-sectional images. The light beam from an OCT system is split by an interferometer into two arms, a sample arm and a reference arm. The sample arm light travels to the sample tissue, then is being reflected, refracted, or absorbed by the tissue and finally travels back to the interferometer. The reference arm is directed to a mirror, which reflects it back to the interferometer, combined with the sample arm light and detected by a detector. The OCT image is built based on the interaction between these two light waves, depending on whether there is constructive or destructive interference between the waves [1]. Cross-sectional images are generated by measuring the delay time and intensity of light reflected or backscattered from internal structures in biologic tissue [1, 3, 4]. There are two types of OCT systems: first generation time domain (TD-OCT) and second generation frequency domain (FD-OCT) (Fig. 1.1) [5, 6]. A reference arm and an interferometer are used by both systems to detect echo time delays of light. While the reference arm in TD-OCT is mechanically scanned by a moving mirror to produce a time-varying time delay, the light source is frequency swept in FD-OCT. As a result, the interference of the light beam from tissue and reference oscillates according to the frequency difference. The main limitation of first TD-OCT systems was the need for an occlusion balloon inflated proximally to the imaged segment to remove blood from the vessel. Since FD-OCT systems use fixed reference mirror and adjustable laser light sources with a wavelength between 1250 and 1370 nm, they can reach a much higher imaging speed, eliminating the need for an occlusive balloon . A summary of physical characteristics of FD-OCT and TD-OCT compared to intravascular ultrasound (IVUS) is shown in Table 1.1. While OCT can produce images with a resolution of 10–20 μm, an order of magnitude higher compared to IVUS, it cannot image through blood, since blood attenuates near-infrared light and therefore clearing of blood from the lumen is required. In addition, the penetration depth of OCT usually ranges between 1 and 3 mm depending on tissue type, which prevents visualizing external elastic membrane in large lesions [7].


Fig. 1.1
Schematic representation of time-domain optical coherence tomography (TD-OCT, left panel) and frequency or Fourier- domain optical coherence tomography (FD-OCT, right panel). (Adapted from Bezerra et al. [5])
Table 1.1
Comparison of IVUS and OCT
IVUS | TD OCT | FD OCT | |
---|---|---|---|
Energy wave | Ultrasound | Near-infrared | Near-infrared |
Wavelength, μm | 35–80 | 1.3 | 1.3 |
Resolution, axial/lateral, μm | 100/200 | 15/90 | 15/20–40 |
Frame rate, frames/s | 30 | 16–20 | 100 |
Pullback rate, mm/s | 0.5–1.0 | 1–3 | 20 |
Axial scans × 1000 | – | 3.2–4.8 | 5.4 |
Lines, axial scans/frame | – | 200–40 | 500 |
Maximum scan diameter, mm | 10 | 6.8 | 9.7 |
Tissue penetration, mm | 10 | 1–2.5 | 2.0–3.5 |
1.2 Equipment
An OCT catheter contains a rotating single-mode optical fiber with a lens and refractor element at its distal end to focus the beam and direct it sideways into the vessel wall [6]. The catheter is connected to a rotary junction with a motor unit to rotate the fiber. Currently available imaging probes for TD-OCT have a maximal outer diameter of 0.019 in. and contain a single-mode fiberoptic core within a translucent sheath. The imaging probes for FD-OCT are integrated in a short monorail catheter comparable to conventional 0.014 in. angioplasty guidewires and 6 Fr or larger guiding catheters. The reflected light signal is detected and converted to digital signals within an OCT console. The console can also be used to control the rotational and pullback speed of the catheter. Finally, it allows adjustment of Z-offset, a variation in the optical path length of the optical fiber within the catheter, by performing calibration before each OCT imaging in order to avoid errors in OCT measurements.
1.3 Image Acquisition and Safety
Similar to the IVUS procedure, patients undergoing OCT imaging require systemic anticoagulation with heparin before the guidewire is inserted into the vessel [6]. In order to avoid catheter-induced vasospasm, image acquisition is performed after administration of intracoronary nitroglycerin. OCT imaging is performed with caution in patients with severely impaired left ventricular function, markedly impaired renal function, a single remaining vessel, or known allergy to contrast media. OCT imaging can be conducted using either manual or motorized pullback. Proper blood clearing during image acquisition is crucial for obtaining good quality images, since infra-red light cannot penetrate blood. There are two blood clearing techniques for TD-OCT, the occlusive and nonocclusive approaches. While the majority of occlusive TD-OCT procedures have been performed using the occlusive method requiring balloon inflation proximal to the lesion, the nonocclusive technique has become a more popular acquisition method with recent improvements in the acquisition speed of TD-OCT. FD-OCT is mostly performed with the nonocclusive flushing technique, which allows reduction of potential myocardial ischemia and vessel injury due to balloon inflation. First, a standard intracoronary guidewire (0.014″) is used to cross the target lesion; then, the OCT probe is positioned over the guidewire distal to the lesion. A pullback is performed manually or automatically (10–40 mm/s) with simultaneous injection of a bolus of contrast agent through the guiding catheter. The infusion rate is usually set to 2–4 mL/s, depending on the artery. Automated contrast injection might help optimize image quality, since the pullback would start automatically only if blood clearance is recognized distally. A proper guiding catheter engagement, coaxially and deeply in the ostium, is important for obtaining high quality images. A manual injection of a small bolus of contrast agent before imaging can be used in order to verify good guide catheter position. Several recent technical developments have dramatically reduced imaging times, resulting in a very short injection sufficient for optimal image acquisition.
In a multicenter registry of 468 consecutive patients who underwent TD-OCT imaging, major complications included five cases of ventricular fibrillation caused by balloon occlusion and/or deep guide catheter intubation, three cases of air embolism, and one case of vessel dissection [8]. There were no cases of coronary spasm or major adverse cardiovascular event during or within the 24-h period following OCT imaging. There were no major complications reported with FD-OCT using nonocclusive flushing in a small feasibility study [9] and a larger single center registry [10], suggesting that FD-OCT is a feasible and safe technique for guidance of coronary interventions. Both occlusive and nonocclusive OCT image acquisition were demonstrated to be safe in several studies [11, 12].
1.4 Image Display and Assessment
The normal vessel wall is characterized by a layered architecture (Fig. 1.2). In the presence of atherosclerotic plaque, the lumen usually appears narrowed, and the layered structure of the vessel wall is deranged (Fig. 1.3). Plaques can be characterized as fibrous, lipid-rich, or calcified, according to plaque characterization criteria developed by histology validation studies [6, 13, 14]. Fibrous plaque appears on OCT imaging as a relatively homogeneous signal-rich region (Fig. 1.3a, b). Calcification is characterized by a signal-poor area with sharply delineated borders (Fig. 1.3c, d). In contrast, lipid-rich plaque appears as a signal-poor region with diffuse borders and an overlying signal-rich layer and a fibrous cap (Fig. 1.4). The low intensity areas with poorly defined outlines correspond to the necrotic core. OCT thin-capped fibroatheroma is a lipid-rich plaque with the minimal fibrous cap thickness less than a predetermined threshold. The most commonly used cutoff of 65 μm is defined by pathology studies [15]. Vessels within the intima may be visualized by OCT as sharply delineated signal-poor voids (Fig. 1.5a). Macrophage accumulation can appear as signal-rich either distinct or confluent punctate regions significantly attenuating the OCT signal (Fig. 1.5b). Cholesterol crystals appear as thin linear signal-rich structures with low signal attenuation (Fig. 1.5c, d). Plaque rupture is defined as a lipid-rich plaque with fibrous cap discontinuity and formation of a cavity inside the plaque (Fig. 1.6a). In contrast, OCT plaque erosion does not show any evidence of cap disruption (Fig. 1.6b). A thrombus is identified by OCT as a protruding mass attached to a luminal surface or floating within the lumen (Fig. 1.7). OCT can differentiate between red cell–rich red and platelet-rich white thrombi. Stent malapposition , underexpansion, dissections, tissue prolapse, and thrombi are the most common immediate findings after stent implantation (Fig. 1.8). OCT can provide unique micron-scale level insights into stent strut coverage for follow-up imaging and characterize the morphology of an in-stent restenosis lesion (Fig. 1.9). An important part of image interpretation is understanding and recognizing OCT imaging artifacts (Fig. 1.10) [5, 6].
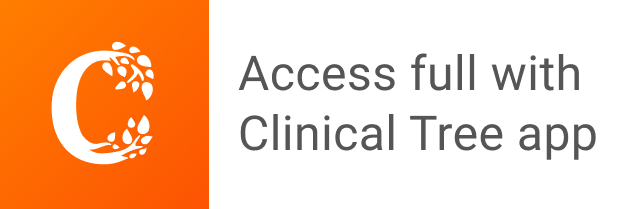