
Introduction
Optical coherence tomography (OCT) is a tomographic imaging technology first described for use in ophthalmology that has been adapted for real-time intravascular imaging. The resolution of OCT is significantly higher than other currently available intravascular imaging modalities, allowing for detailed characterization of the morphological features of coronary arteries, coronary plaques, and intracoronary stents. OCT is analogous to intravascular ultrasound (IVUS) with light as the energy source instead of sound. Light is directed at a target, and the magnitude and echo time delay of the backscattered light signal is measured. Because it utilizes light, OCT has a resolution that is an order of magnitude greater than IVUS. Tissue-level properties can be assessed, allowing for differentiation of tissue types based on their optical characteristics. Higher resolution imaging also allows for more accurate assessment of vascular lumen size, vascular pathology, presence of thrombus, coronary stent strut apposition after percutaneous coronary intervention (PCI), and vascular response to previously placed coronary stents. Because of significant blood attenuation of light, OCT imaging requires a blood-free zone for imaging. First generation OCT imaging systems utilized an occlusive balloon with saline flush through a distal balloon lumen. Second generation OCT systems, utilizing a nonocclusive contrast flushing method to create a blood-free zone combined with faster catheter pullback, have significantly simplified image acquisition. These improvements allow for expansion of OCT use beyond research to more routine use to assess intravascular pathology and to guide PCI.
Physics of Optical Coherence Tomography
OCT utilizes optical interference of near-infrared light to generate images. Near-infrared light is emitted from a distal tip of an optical fiber located within the imaging catheter and directed at the target tissue ( Figure 17-1 ). When light encounters a boundary between objects with varying optical impedances, a portion of the light is backscattered. The OCT catheter measures the magnitude and echo time delay of the backscattered light signal. To allow for recording of the reflected light, an interferometer is utilized to combine the reflected light from the sample with a reference beam reflected off of a reference mirror at a known distance. The summed beams from the sample and the reference mirror are then measured by the detector. The first generation time domain OCT (TD-OCT) systems utilized a broadband light source and determined tissue depth by changing the distance to the reference mirror. Because of the need for mechanical sweep of the reference light source, the rate of image acquisition in TD-OCT is inherently limited. Maximum pullback speeds with TD-OCT systems are approximately 2-3 mm/second. In the context of need for a blood-free field, this limits the length of coronary artery that can be imaged during one pullback of the imaging catheter. In second generation Fourier-domain OCT (FD-OCT) systems, the light source is monochromatic and emits various wavelengths between 1250 nm and 1350 nm in a continuous sweep. Fourier transformation of the interference signal generated allows for calculation of the reflections returning from different depths. As result, there is no need for mechanical adjustment of the reference light path. This allows all echo time delays to be measured simultaneously, leading to significantly faster image acquisition. FD-OCT systems allow pullback speeds of the imaging catheter of 20 mm/second.

Because light is the energy source, tissue can be imaged with an axial resolution of 10 µm and lateral resolution of 20 µm. Tissue penetration is limited to a depth of 2 mm but varies significantly depending on the imaged tissue. Lipid, which is high attenuating, allows for significantly less tissue penetration than collagen or calcium, which are low attenuating. In comparison IVUS, which is currently the most commonly used intravascular imaging modality, provides a resolution of 150-250 µm to a depth of up to 10 mm.
Image Acquisition by Optical Coherence Tomography
Time Domain Optical Coherence Tomography
The first OCT used clinically was the M2 and M3 TD-OCT system manufactured by LightLab (Westford, Massachusetts, now part of St. Jude Medical). In the M2/M3 systems, a console contains the pullback device; the optical imaging components including the light source, beam splitter, reference arm, and detectors; and a computer for image creation. Images are recorded by a fiber-optic wire that rotates inside a protective sheath. The wire is attached to an automated pullback engine integrated with the console. An over-the-wire low-pressure occlusion balloon catheter with distal flush ports is used to occlude the imaged vessel at a low-pressure inflation (0.5 atm) and infuse saline or lactated Ringer’s at approximately 0.5-1.0 mL/s to displace blood during imaging acquisition. Per manufacturer recommendations, the occlusion time should be limited to 30 seconds. Images are obtained during pullback at a rate of 0.5-2.0 mm/s. There are significant limitations from the occlusive technique for blood displacement, specifically transient ischemia, inability to image proximal segment of vessel due to balloon occlusion, and the complexity and time-consuming nature of the procedure. A nonocclusive technique for blood removal is also possible. In this approach, blood is displaced by continuous injection of iso-osmolar contrast or mixture of dextran and lactated Ringer’s through the guiding catheter by manual injection or automated injection. For automated injections, the volume of injection should be 50 mL at a rate of 1.5-3 mL/s depending on vessel size. Pullback is then performed at a speed of 2-3 mm/s during contrast injection. The nonocclusive technique for blood displacement has a safety profile similar to the occlusive technique.
Frequency Domain Optical Coherence Tomography
The first commercially available OCT system in the United States was a frequency domain (FD)-OCT system, C7XR OCT manufactured by St. Jude Medical. In this second generation platform, the optical probe is integrated into a delivery catheter with a profile of 2.7 Fr and length of 140 cm. The catheter has a rapid exchange monorail tail compatible with standard 0.014 coronary wires and can be delivered via a 6 Fr guide catheter. Radio-opaque markers, identifying the distal tip, location of imaging lens, and 50 mm proximal to the lens allow for alignment of the catheter with the vessel segment of interest. During the pullback the optic fiber probe is pulled along the catheter sheath. As with the first generation TD-OCT systems, the FD-OCT systems contain a dedicated pullback device and a console that processes and stores the data. The C7 FD-OCT systems can acquire images at a pullback speed of 20 mm/s, allowing for imaging of a 50-mm vessel segment in less than 3 seconds. The newly available ILUMIEN OPTIS imaging system (St. Jude Medical, Minneapolis, Minnesota) allows for imaging of a 75-mm segment of vessel at a pullback speed of 40 mm/s. Because of the rapid pullback in these systems, adequate blood displacement can be achieved with a single bolus injection of contrast. Lactated Ringer’s can also be used to minimize contrast load in patients with renal impairment. Guiding catheter should be positioned coaxially with vessel ostium to maximize delivery of contrast for blood clearance. The manufacture protocol recommends a 14-mL injection of contrast at a rate of 4 mL per second. The technique for image acquisition is significantly simplified and faster with the C7 and OPTIS system compared with the first generation M2/M3 systems.
Potential Risks and Complications
Overall, the technique appears to be safe with 0.2% incidence of vessel dissection due to the imaging system and 1% incidence of ventricular fibrillation secondary to balloon occlusion, when the first generation TD-OCT system was used. Sinus bradycardia, tachycardia, and atrioventricular block have also been reported in the context of balloon occlusion but are rare. Blood clearance during OCT image acquisition can produce transient ischemia especially with the occlusive technique. This is of particular concern in patients with single-vessel myocardial blood supply. Chest pain and transient ST-segment elevation during vessel occlusion are common, occurring in about 50% of patients. Risks of transient ischemia are significantly reduced with the second generation rapid pullback systems. Coronary spasm and coronary dissection are potential complications whenever any device is introduced into a coronary artery and likewise can occur during OCT imaging. In order to reduce the risk of coronary spasm, intracoronary nitroglycerin should be administrated prior to image acquisition. The incidence appears to be less than 1% for either spasm or dissection. Similarly, there is a risk of air embolism and thrombus injection as would occur with any other intracoronary procedures. Complication rates with OCT are similar to those seen with IVUS in the PROSPECT study. Fastidious attention to flush lines, contrast injection, and wire management is required as with any procedure. Anticoagulation prior to intracoronary introduction of OCT system is mandatory.
Evaluation of Coronary Pathology
Normal Artery
By histology, the normal coronary artery has three tissue layers: intima, media, adventitia separated by an internal and external elastic lamina. All three layers can by visualized by OCT in a normal artery ( Figure 17-2 ). The innermost adluminal intimal layer is seen by OCT as a signal-rich thin band. The media is immediately below the intima and appears as an area of low signal intensity surrounded by the signal-rich adventitia. OCT assessment of intimal thickness is highly accurate, allowing for detection of the earliest marker of atherosclerotic disease, intimal thickening secondary to deposition of lipids. Coronary plaques are typically identified by the presence of focal thickening and loss of the normal three-layered structure of the coronary artery. Mature coronary plaques are generally classified by histology as lipid-rich, fibrous, or fibrocalcific based on their tissue compositions, each of which can be distinguished by its OCT appearance. In cadaveric studies of human arteries with histology as the gold standard, OCT has sensitivity for lipid plaque, fibrous plaque, and calcified plaque of 95%, 98%, and 100%, respectively. The specificity of OCT for the same three plaque tissue components was 98%, 94%, and 100%, respectively. These results compare favorably to both integrated backscatter IVUS and conventional grayscale IVUS.

Lipid Plaque
Lipid plaque is defined by the presence of signal-poor areas with diffuse borders resulting from backscatter and rapid attenuation from the lipid-containing region located below a fibrous cap ( Figure 17-3A ). The fibrous cap is typically a homogeneous signal-rich band overlying the signal-poor lipid core. The minimal thickness of the fibrous cap is critical for identifying thin-cap fibroatheromas (TCFAs).

Fibrous and Fibrocalcific Plaque
Fibrous plaques are visualized on OCT as homogeneous areas with high reflectivity and low attenuation ( Figure 17-3B ). Calcification within the coronary artery is demarcated by a signal-poor region with sharp edges and low attenuation ( Figure 17-3C ). This is distinct from lipid pools that are signal-poor areas with diffuse borders and high attenuation. Because both calcium and lipid generate low backscatter, distinguishing calcified plaque from lipid-rich plaque can at times be difficult to untrained interpreters.
Intraluminal and Intramural Pathology Seen by Optical Coherence Tomography
Macrophages
Increased inflammation and macrophage infiltration is a hallmark of vulnerable plaques. OCT can indirectly assess for macrophage density within vulnerable plaques on the basis of signal variance within the raw OCT data. Fibrous caps containing macrophages have significant OCT signal variance, which when normalized for variations in OCT system settings to generate a normalized standard deviation (NSD) of the OCT signal, can be used to identify areas with significant macrophage infiltration. When the data are compressed to generate images, areas of macrophage infiltration show a granular pattern with heterogeneous back shadow ( Figure 17-3D ). Autopsy studies of lipid-rich plaques have shown that OCT can identify plaques with greater than 10% macrophage density within the fibrous cap (identified by CD68 positivity with immunohistochemistry) with near 100% sensitivity. OCT studies of patients presenting with acute coronary syndrome (ACS) have shown significantly greater macrophage density within fibrous caps compared with patients with stable angina. Sites of plaque rupture appear to have the highest density of macrophage infiltration. Although intriguing, the ability to identify macrophages in vivo using signal variance has not been fully validated and further studies will be required to confirm.
Cholesterol Crystal
Cholesterol crystals are identified by the presence of liner signal streaks typically in the context of a lipid-rich plaque ( Figure 17-3E ). The accuracy of OCT for the detection of cholesterol crystals has not been validated by histology.
Microchannels
OCT can detect within coronary plaque microchannels that appear as tubuloluminal structures with no signal inside ( Figure 17-3F ). These are believed to represent neovascularization and are found in higher abundance in thin capped lipid plaques and in patients presenting with plaque rupture. The presence of microchannels has also been associated with plaque progression.
Thrombus
Thrombus is identified by the presence of an irregular mass either attached to the vessel wall (mural thrombus) or free within the vessel lumen. Platelet-rich white thrombus is a homogeneous signal-rich mass with low attenuation resulting in minimal shadowing, whereas red thrombus is a mass with rapid attenuation resulting in significant shadowing ( Figure 17-4A, B ). The rate of signal attenuation, specifically a half attenuation width of 250 µm, can distinguish red and white thrombi with a sensitivity of 90% and specificity of 88%.

Others
In addition to coronary plaques, acute coronary syndrome (ACS) can be caused by spontaneous coronary artery dissection and vasospasm. OCT is sensitive for detecting and characterizing coronary dissections and may be useful in guiding interventions to treat the dissection ( Figure 17-4C ). The presence of double-lumen, dissection flap or intramural hematoma can be readily visualized by OCT even when angiographically not obvious. The morphological changes, specifically medial contraction and intimal gathering, that underlay coronary vasospasm have also been visualized by OCT.
Thin-Cap Fibroatheroma
Coronary artery disease is the leading cause of death worldwide, most often by precipitating an ACS. ACS is an acute manifestation of the chronic process of atherosclerosis. As a result, there has been significant interest in understanding the mechanism by which coronary artery disease that is otherwise asymptomatic can lead to a sudden myocardial infarction. The term “vulnerable plaque” was initially coined in reference to coronary stenosis that did not appear to be significant by angiography but subsequently caused acute myocardial infarction. Vulnerable plaques can be present in any location within the coronary arteries but are most commonly found in the proximal portion of the three main coronary vessels often at or near bifurcations. ACS most frequently occurs when a vulnerable plaque ruptures, exposing the thrombogenic contents of the plaque to blood. Vulnerable plaques that are at high risk of precipitating an acute coronary event appear to have several histologic features that distinguish them from more stable coronary plaques. Autopsy studies have identified the presence of thin fibrous caps (<65 µm), large lipid cores (more than 40% of the overall plaque volume), and increased infiltration of macrophages into the plaque cap as the most common features of high-risk coronary plaques. In addition, several other features have been linked to vulnerable plaques including positive remodeling of the affected vessel, increased vasa-vasorum neovascularization, and intraplaque hemorrhage. Because of a resolution capability of 10 µm, OCT is the only current modality that can readily identify and measure the thin fibrous caps of vulnerable plaques. Accurate and reproducible measurement of fibrous cap thickness is critical for the accurate identification of thin-cap fibroatheroma (TCFA). The typical method is averaging the measurement of cap width at several sites of minimal cap thickness based on visual estimation ( Figure 17-5 ). Thickness is measured from the coronary artery lumen to inner border of the signal-poor region that identifies the lipid pool. In analyzing ruptured plaques, it can be challenging to identify the fibrous cap and accurately measure its thickness due to the presence of associated thrombus. Studies have either focused on the fibrous cap thickness at the preserved portions of the cap, which can overestimate cap thickness, or measured the remnants of the cap at the rupture site. A three-dimensional volumetric method has been proposed for more accurate measurement of fibrous cap area and thickness but has not been validated. In a cadaveric study, OCT measurement of fibrous cap correlated well with histological measurements (r = 0.90). Published studies have varied in their definition of OCT defined TCFAs both in the cutoff for defining a cap as thin (65 µm vs. 70 µm vs. 80 µm) and the minimum lipid burden within the coronary plaque.

Pitfalls of Image Interpretation
Recognition of potential artifacts and limitations of OCT are crucial in proper interpretation of images. Incomplete clearance of blood is one of the most common causes of artifact during OCT imaging. Residual blood attenuates the light beam resulting in limited visualization of the vessel ( Figure 17-6A ). Significant blood attenuation can hamper accurate measurement of vessel size and can easily be confused for thrombus. With OCT, both lipid and calcium appear as signal-poor areas during OCT image acquisition. Lipid-rich plaques have indistinct borders while calcium-rich plaques have sharp borders surrounding the signal-poor areas. While these differences allow for distinguishing the two plaque types, there remains a significant overlap and potential to mischaracterize lipid-rich and calcium-rich plaques. Because of the limited tissue penetration of OCT to 2-3 mm, determining the composition of thick plaques can become problematic with increasing signal drop-off. Attenuation of OCT signal deep within the artery wall can be confused for lipid pool and alternatively deep lipid can be misinterpreted as signal attenuation. Similarly, lack of tissue penetration prevents accurate measurement of total plaque burden and precludes assessing for vessel remodeling.

Optical Coherence Tomography Imaging Artifacts
- •
Shadowing is a result of signal drop-off distal to an object such as guidewire ( Figure 17-6B ), stent strut ( Figure 17-6C ), blood within the vessel or within the imaging catheter, macrophages, and thrombus. As result of signal loss, the underlying vessel structure cannot be visualized.
- •
Tangential signal dropout occurs when a catheter is nearly adjacent to a vessel wall, resulting in light being directed almost parallel with the vessel surface. As a result, the light source is significantly attenuated and there can be significant signal drop-off below the vessel lumen surface in the area immediately adjacent to the imaging catheter. It is important not to confuse this artifact with pathologic causes of signal-poor regions within vessel walls such as lipid-rich plaques.
- •
Nonuniform rotation distortion arises as a consequence of binding of the rotating optical components during image acquisition, typically due to a defective catheter or during imaging of tortuous or calcified vasculature or through a narrow stenosis. It appears as a smearing of the OCT image in the circumferential direction.
- •
Saturation artifact ( Figure 17-6D ) is caused by objects with highly reflective surface causing such high backscatter that they cannot be detected accurately. This results in linear streaks along an axial direction. Saturation artifact is typically caused by stent struts, guidewires, and occasionally by tissue surface.
- •
Motion artifact is caused by movement of the artery with respect to the imaging catheter ( Figure 17-6B ). It is a more significant problem with the TD-OCT systems that have a slower pullback speed and therefore acquire images over significantly more cardiac cycles. Seam lines, which are axial discontinuities, can arise when the imaging catheter moves relative to the vessel lumen during the acquisition of a single cross section. Movement of the artery can also disrupt the pullback, resulting in repeated images of the same anatomic area.
- •
Fold-over artifacts are specific to FD-OCT systems. They occur when the vessel lumen is larger than the imaging depth of the OCT system.
- •
Merry-go-round and sunflower effect are caused by eccentric wire positions that result in a light beam that is not perpendicular relative to the artery wall ( Figure 17-6C ). Lateral resolution in the wall distal to the position of the wire is reduced (“merry-go-round effect”) and the reflection of stent struts aligns toward the location of the wire, making it appear as if the stent struts are bending toward the wire (“sunflower effect”).
Assessment of Coronary Plaque Characteristics
Clinical Presentations and Plaque Morphology
The danger of TCFAs arises from their propensity to rupture and precipitate an acute coronary event. OCT identifies the presence of lipid-rich culprit plaques in 90% of patients presenting with ST-elevation myocardial infarction (STEMI) and 75% of patients presenting with non-ST-elevation myocardial infarction (NSTEMI). This compared to 60% of patients presenting with stable angina. ACS patients also have thinner fibrous caps, large lipid burden, increased number of plaques with macrophages, and significantly more OCT-defined TCFAs (64.7% vs. 14.9%, p < 0.001). Furthermore, patients with STEMI have a higher prevalence of OCT-defined TCFAs compared with patients with NSTEMI. Ruptured plaques are seen more frequently in patients presenting with ACS compared with stable angina.
One of the challenges of treating patients with coronary artery disease is recognizing plaques that are likely to progress with the goal of identifying patients who would benefit from either more aggressive pharmacological treatment or prophylactic intervention. Studies involving small cohorts of patients with nonobstructive disease have identified TCFA and microchannels seen by OCT as potential predictors of plaque progression. Specifically, plaques that had significant progression over a period of 7 months were significantly more likely to be TCFA or to have microchannels at baseline. Further prospective studies involving larger numbers of patients are needed to determine if these findings are significant enough to drive clinical decision making.
Etiology of Acute Coronary Syndrome
There are believed to be three principal mechanisms for acute coronary events leading to sudden cardiac death: rupture of thin-capped fibroatheromas, erosion of fibrous caps, and protruding calcific nodules ( Figure 17-7 ). Plaque rupture is defined by the presence of a fibrous cap discontinuity resulting in a cavity formation with communication between the cavity and the coronary artery lumen ( ). By pathology, plaque erosions are identified by loss of the endothelial lining with overlying thrombus. In a study comparing IVUS, angioscopy, and OCT, OCT was significantly better at detecting superficial plaque erosions. With a resolution of 10-15 µm, OCT cannot visualize the endothelial lining of coronary arteries. Consequentially, surrogate markers must be utilized to identify plaque erosion by OCT. Erosions can potentially be identified by the presence of an intact fibrous cap with attached superficial thrombus or by the presence of an intact fibrous cap with irregular surface in the absence of superficial thrombus. The presence of significant intracoronary thrombus, by limiting visualization of the underlying plaque and potentially hiding the presence of a fibrous cap discontinuity, significantly impairs the ability to distinguish plaque erosion from plaque rupture. Protruding calcific nodules have been defined by the presence of convex-shaped calcium that is covered by a thin fibrous cap or fully exposed to the lumen. Fracture of calcific plate into the lumen frequently forms sharp angles which can be readily identified by OCT.

A study of patients presenting with ACS identified plaque erosions at the culprit lesion in 31.0% of cases, the majority in patients presenting with NSTEMI. In contrast, plaque rupture was seen at the culprit site in 43.7% of patients, predominantly in patients with STEMI. Calcified nodules were causal in 7.9% of patients. Patients with plaque erosions are typically younger and appear to have higher levels of inflammatory markers compared with those with plaque rupture. Vergallo et al. further showed that patients presenting with coronary plaque rupture at the culprit lesion were more likely to have ruptured plaques at nonculprit sites than patients presenting with ACS caused by erosion. These results suggest that patients presenting with plaque rupture represent a subset of patients with increased pancoronary vulnerability. Distinguishing plaque erosion from plaque rupture may have therapeutic significance. Evaluation of patients’ post-thrombolysis by OCT showed significantly more residual thrombus at sites of plaque rupture with a core of platelet-rich white thrombus surrounded by large amounts of red thrombus. In contrast, sites of plaque erosion had significantly less thrombus that was predominantly platelet rich. Prati et al. analyzed 31 patients presenting with STEMI who were found to have an intact fibrous cap after aspiration thrombectomy. Twelve patients with subcritical stenosis were treated with dual antiplatelet therapy and no stenting. At median 2 years’ follow-up, all patients remained asymptomatic. This proof of concept study suggests an alternative treatment strategy forgoing stenting for patients with ACS and OCT verified non-obstructive plaque erosion may be possible.
Acute Myocardial Infarction at Rest versus with Exercise
The location of plaque rupture appears to correlate with the clinical scenario. Plaque rupture at the proximal portion of the plaque appears to occur more commonly with STEMI, while plaque rupture at the distal end of the plaque is seen more frequently with NSTEMI. A study of culprit lesions in patients presenting with ACS revealed plaque rupture at the mid portion of the plaque correlates with the onset of coronary event at rest while plaque rupture at the plaque shoulder typically associates with coronary events occurring during activity. The thickness of the ruptured fibrous cap in patients with ACS brought on by exertion was significantly higher than in patients with ACS at rest. Of note, in a significant minority of patients, the fibrous cap was thicker than the 65 µm used to define TCFAs.
Role of Optical Coherence Tomography In Percutaneous Coronary Intervention
Pre-stent Deployment
The resolution of OCT makes it a potentially powerful tool to guide percutaneous coronary intervention (PCI). Accurate assessment of reference vessel size and lesion length are keys to appropriate stent sizing. Current OCT platforms contain software for semiautomatic vessel contour detection allowing for rapid, accurate, and reproducible measurements of reference vessel diameter and the minimal luminal diameter of the target lesion. The length of the lesion can also be measured rapidly in manual fashion. The potential exists for fully automated definition of luminal boundaries allowing for precise measurement of minimal lumen areas throughout the vessel segment analyzed. The most recent OCT system (ILUMIEN OPTIS OCT, St. Jude Medical) catheter can acquire images over a longer segment of vessel (75 mm) at a faster pullback rate (40 mm/s). It is also reported to have a higher resolution then the previous generation system. The console allows for fully automated analysis of lumen contour in order to measure lesion length and reference vessel diameter. Using fractional flow reserve (FFR) as the gold standard, OCT is moderately accurate in determining lesion severity with similar accuracy to IVUS. Minimal luminal areas of 1.91 mm 2 and percent lumen area stenosis >70% appear to be the best cutoff values to identify lesions with FFR <0.75. Although OCT is sensitive for identifying severe lesions, the lack of specificity limits the positive predictive value of OCT-defined severe lesions. Lesion characteristics such as fibrous, lipid, and calcium content, as defined by OCT, correlate with risk of post-procedure complications. The presence of thrombus can also readily be identified prior to intervention. A recent randomized study evaluated the role of OCT in guiding in-stent thrombus removal for patients presenting with ACS and found significantly reduced thrombus volume in-stent and significantly larger stent area in the OCT-guided group.
Immediate Post-stent Deployment
A key component of a successful intervention is adequate stent expansion resulting in a well-apposed stent ( Figure 17-8A and ). Metal stent struts are highly reflective and generate a strong signal with shadowing behind the stent strut (“blooming”). Bioabsorbable stents with polymeric struts, in contrast, do not cause shadowing. Absorbable stents with metallic scaffolds appear similar to typical metal stents at implantation but overtime become less reflective and lose the blooming effect. Based on IVUS definitions for stent expansion, adequate stent expansion is defined by OCT as a minimal in-stent lumen area that is >90% of the reference vessel lumen area. In the setting of significant artery tapering, adequate stent expansion is defined by a minimal in-stent area that is greater than 100% of the luminal area of the small distal reference segment. Inadequate stent expansion leading to stent strut malapposition ( Figure 17-8B ) increases the risk of stent failure: either restenosis or in-stent thrombosis.
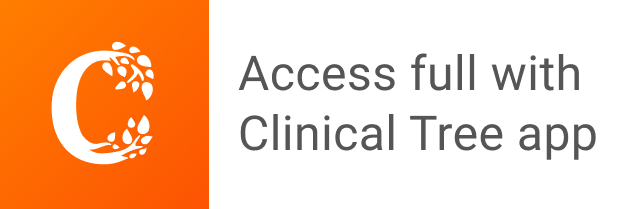