Direct
Indirect
Pulmonary infections
Sepsis
Inhalations
Multiple trauma
Pulmonary contusions
Blood transfusion
Aspiration
Severe burns
Mechanical ventilation
Pancreatitis
Major surgery
Ischemia-reperfusion injury
Significant overlap exists between the regulation of normal postnatal lung growth and development and clearance of alveolar fluid, apoptosis, innate immunity, early inflammatory responses to mechanical ventilation, as well as repair mechanisms in the lungs [12, 13]. The design and interpretation of existing and future studies investigating mechanisms of lung injury and repair should include consideration of the possibility that there are age-dependent differences.
Exudative Phase: Acute Alveolitis
This phase is characterized by disruption of the alveolar epithelial-endothelial barrier by injury to alveolar epithelial cells, pulmonary capillary endothelial cells, or both. The large surface area and thin structure of the alveolar unit – consisting of the unique structure of alveolar epithelial and pulmonary capillary endothelial cells sharing a common basement membrane – is efficient for gas exchange but makes it susceptible to injury. The pathobiologic processes of this phase are the rapid accumulation of a proteinaceous fluid (exudate) and infiltration of activated leukocytes into the alveolar airspace, reduced production and/or inactivation of surfactant, coagulopathy, activation of apoptotic pathways, and initiation of fibrosis [8, 14, 15].
Direct Epithelial Injuries
Early pathologic descriptions of diffuse alveolar damage (DAD) suggested a predominance of alveolar epithelial cell injury [16]. Injury to alveolar epithelial cells results in loss of surfactant production, decreased alveolar fluid clearance, and exposure of the shared pulmonary epithelial-endothelial basement membrane, further activating inflammatory and coagulation cascades.
Infectious pathogens and aspiration are the most common causes of direct alveolar epithelial injuries [17–19]. Pathogens may cause alveolar cell necrosis, apoptosis, or pyroptosis [20–22]. Alveolar epithelial cell necrosis and pyroptosis cause uncontrolled release of damage-associated molecular patterns (DAMPs) as well as pro-inflammatory cytokines [23–26].
Mechanical ventilation is the life-saving support for patients with ARDS. The earliest observations of patients with ARDS suggested that adding positive end-expiratory pressure (PEEP) improved survival, and limiting tidal volume remains the single most significant improvement to the care of these patients [27, 28]. However, the converse is also true in that mechanical ventilation may also worsen lung injury [29]. Ventilator-associated lung injury (VALI) describes the potential contribution of mechanical ventilation to patients with existing lung injury, whereas ventilator-induced lung injury (VILI) is used to describe injury directly caused by mechanical forces. Since many carefully designed laboratory studies have established multiple mechanisms by which the mechanical forces imposed on the respiratory system causes injuries in the lungs, VILI will be used throughout the rest of this chapter. Ventilator-induced lung injury may occur by shear stretch (volutrauma), repeated opening and closing of atelectatic lung (atelectrauma), production of pro-inflammatory cytokines via mechanotransduction (biotrauma), and oxidative stress [30, 31]. Barotrauma is a term that continues to be used to describe lung injury resulting in air leaks (pneumothorax, pneumomediastinum, etc.) as well as lung injury associated with high airway pressure. However, barotrauma is likely somewhat of a misnomer as an elegant study by Dreyfuss and colleagues showed that pressure, in the absence of high absolute lung volume, did not cause lung injury [32].
The apparent discrepancy between volutrauma and barotrauma in the ARDS patient with significant amounts of derecruited lung and high airway pressures can be reconciled by recognizing that, in heterogeneous lung disease, the energy of each tidal volume is delivered to only [33, 34]. Amato’s work showing that “driving pressure” was the variable that most stratified risk is consistent with the concept that mechanical power is the sum of the forces needed to recruit atelectatic and stretch inflated regions, divided by the time in which those forces are applied [35–37]. PEEP and prone positioning have potential to recruit atelectatic regions (preventing atelectrauma) and thereby reduce the fraction of tidal stretch delivered to individual lung regions by increasing the total amount of inflated lung [38, 39]. This is the most likely physiologic rationale for studies that have shown improved outcomes in adult and pediatric patients with ARDS treated with higher PEEP [40, 41]. However, if additional PEEP does not recruit atelectatic regions, then the remaining lung regions will rest at higher levels of inflation at end inspiration, and the fractional tidal stretch will be increased – worsening volutrauma [42]. Although high-frequency oscillatory ventilation (HFOV) has potential to provide improved recruitment with a constant high mean airway pressure and avoid volutrauma by delivering ultralow, subphysiologic dead space tidal volumes, the potential to cause VILI with the oscillator should now be apparent. The frequency of HFOV is inversely related to convective tidal volume, and the time in which that tidal volume is delivered is very short, making the potential for the power delivered to regions of inflated lung to be high [37, 43–45].
There are many benefits to having patients breathe spontaneously while treated with mechanical ventilation, but high amounts of power, causing worse direct injury to the lung, may be difficult to discern during spontaneous breathing [46]. In order to reduce the power delivered to the lung, there was early recognition that hypercapnia should be permitted [47]. Although data suggest that hypercapnia may even be therapeutic, patient dyspnea from respiratory acidosis remained a substantial barrier to effective implementation of “lung-protective ventilation” in many academic centers [47, 48]. Significant negative inspiratory forces generated at a high rate by the dyspneic patient with lung injury are likely to contribute to “VILI” [46, 49]. Treatment of ARDS patients with neuromuscular blockade has been shown to reduce biomarkers of alveolar epithelial and endothelial injury as well as reduce mortality [50–52]. There remains much controversy surrounding the use of extracorporeal life support for the treatment of patients with ARDS, but it is likely the only way to truly “rest” the lungs [53–59]. The nuances of “rest settings” are beyond the scope of this chapter.
Indirect Lung Injury
Indirect lung injury refers to injury to or activation of the vascular endothelium. Sepsis is the commonest cause of indirect lung injury (Table 3.1) [17, 18]. However, injury to the pulmonary endothelium may also be a direct injury. The effect of mechanical stretch is often considered within the scope of injury to the alveolar epithelium, but a large body of data suggests that VILI includes the pulmonary endothelium [60]. Mechanical, chemical, and cellular injuries to the pulmonary endothelium cause alveolar barrier dysfunction, activate inflammatory and coagulation cascades, change pulmonary vascular resistance, and lead to multiorgan dysfunction [61, 62]. Elevated pulmonary vascular resistance and thrombosis of pulmonary capillary beds can increase alveolar dead space. Biomarkers of endothelial activation and injury have been widely studied, and several have been associated with outcomes in children and adults with ARDS [8, 62–64]. An animal model of endothelial injury suggests that age-dependent differences in endothelial permeability are due to differential regulation of adherens junctions between endothelial cells [65].
Angiopoietin 1 (Ang-1) and angiopoietin 2 (Ang-2) are important endothelial growth factors that function in opposition via Tie2 receptors on endothelial cells [66, 67]. Ang-1 is a Tie2 agonist and is highly important to normal, quiescent, endothelial barrier function via cytoskeletal reorganization and increased VE-cadherin at inter-endothelial junctions. Ang-1 stimulation increases endothelial barrier function and thereby reduces tissue edema in multiple infectious and inflammatory models [66, 67]. Ang-2 competitively inhibits Ang-1-Tie2 binding and is released by endothelial cells during inflammatory states. Ang-2 neutralization and antibody clustering has reduced mortality and organ failure in laboratory models of severe infections [66]. The ratio of Ang-2/Ang-1 in plasma has been associated with mortality in adults with ARDS and high dead space fractions, and plasma Ang-2 was associated with mortality in critically ill adults and children with ARDS [68–70].
The endothelial surface layer (ESL) is a glycocalyx comprised of an apical extracellular matrix of glycoproteins, proteoglycans, and glycosaminoglycans (GAGs) creating an organized carpet-like layer between the endothelial cell membrane and the capillary lumen [71, 72]. Advances in understanding the dynamic structure and function of the glycocalyx were limited by tissue fixation techniques until intravital microscopy techniques allowed visualization in living animals [73, 74]. The glycocalyx contributes to fluid and molecular permeability of the endothelial barrier, transduces vascular shear stress, and regulates leukocyte-endothelial adhesion and platelet activation [73, 74]. The pulmonary glycocalyx appears to be significantly thicker (1.5 μm vs 0.5 μm) as compared with some vascular beds but may occupy less of the lumen as compared with the heart or kidney [75–78].
Glycocalyx fragments are found in adult patients with septic shock, and mounting evidence supports that disruption of the glycocalyx is an important mediator of the pathophysiology of sepsis [73, 79]. Heparan sulfate is one of the GAGs in the glycocalyx and contributes to the regulation of endothelial barrier dysfunction, mechanotransduction of shear stress-induced vasoreactivity, and leukocyte adhesion [74]. In a murine model of sepsis, pulmonary endothelial cells released activated heparinase, cleaving heparan sulfate, thinning the ESL, and exposing endothelial adhesion molecules for neutrophils [76]. Elevated plasma levels of heparan sulfate has also been shown in adult patients with lung injury from sepsis and pancreatitis, but not from bacterial pneumonia [80]. In a sepsis model of lung injury, mice treated with intraperitoneal lipopolysaccharide (LPS) showed disruption of the 3-dimensional glycocalyx structure, increased plasma levels of glycocalyx components (syndecan-1) and thrombomdulin, increased permeability of pulmonary capillaries, and activated neutrophils binding and traversing pulmonary endothelial cells [78]. However, not all studies of disruption of the glycocalyx are consistent with changes in vascular permeability. In an experimental rat model of nontraumatic hemorrhagic shock, endothelial degradation and shedding of glycocalyx occurred without evidence of increased vascular barrier permeability [81].
Neutrophil reactive oxygen species, supplemental oxygen, and altered endothelial nitric oxide signaling may contribute to elevated pulmonary vascular resistance. Pulmonary endothelial angiotensin-converting enzyme (ACE) and ACE2 genetic polymorphisms and activity correlate with mortality in children and adults with ARDS [8, 82]. ACE and ACE2 are another mechanism by which pulmonary endothelial biology, pulmonary vascular resistance, inflammation, apoptosis, and coagulation may intersect in the pathobiology of ARDS [83].
Alveolar Fluid Clearance
Injuries to the alveolar epithelial-endothelial barrier results in increased permeability and disruption of alveolar fluid clearance (AFC), leading to pulmonary edema. Accumulation of pulmonary edema fluid reduces respiratory system compliance and impairs gas exchange (ventilation/perfusion mismatch and shunt). The integrity of the alveolar epithelial-endothelial barrier requires maintenance of a thin layer of alveolar wall liquid (AWL) coating the alveolar epithelium. The AWL is necessary for the dispersion of surfactant and is dependent on regulated flow of water, proteins, and small solutes across postcapillary venules into the alveolar airspace [84]. Excess alveolar fluid is removed by sodium-dependent transport by type II alveolar epithelial cells [85].
Activation of the pulmonary endothelium results in increased permeability by both para- and transcellular pathways [86]. The endothelial glycocalyx is highly hydrated and regulates large molecular permeability into it, making the “Starling principle” an inadequate explanation of the forces regulating flow of fluid across endothelial barriers [77, 87–89]. Furthermore, the dynamic structure of the glycocalyx makes modeling flow across the barrier even more complex [88]. Injury to the pulmonary endothelial glycocalyx is likely to be an important mechanism of increased alveolar permeability, and restoration of the glycocalyx is associated with resolution of pulmonary edema [78].
The rate of AFC has been associated with mortality in adult patients with ARDS [90]. Epithelial sodium channels (ENaC), the cystic fibrosis transmembrane conductance regulator (CFTR), Na+-K+-ATPase, and aquaporins (cell membrane water channels) are involved in the clearance of fluid from the distal airspaces into the interstitium of the lung. Salt and water transport is regulated by catecholamines, glucocorticoids, mineralocorticoids, thyroid hormone, growth factors (epidermal growth factor (EGF), transforming growth factor alpha (TGFα), keratinocyte growth factor (KGF), or fibroblast growth factor 7 (FGF-7)), fibroblast growth factor 10 (FGF-10), nuclear factor kappa-B (NFκB), serine proteases, and Fas/FasL, although therapies targeting AFC have not improved outcomes in adults with ARDS [85, 91–93]. Regulation of postnatal lung development may be a protective factor in children with ARDS, in part through preservation of AFC [13]. Age-dependent differences in alveolar epithelial-endothelial barrier function have been shown in mice treated with intraperitoneal lipopolysaccharide [65].
Male gender has been associated with lower alveolar fluid clearance in adult patients with ARDS, whereas premenopausal women are more likely to have high alveolar fluid clearance. These findings are supported by animal data showing that progesterone and estrogen increased expression and function of the epithelial sodium channel [90, 94]. Beta-adrenergic agonists upregulate alveolar fluid clearance in human lungs [95]. However, two randomized placebo-controlled trials of treatment with intravenous salbutamol did not show a reduction in ventilator-free days or mortality in adults with ARDS [96, 97]. Animal studies of KGF therapy for acute lung injury have suggested that pre- but not postinjury treatment is protective [98]. A double-blind, randomized, placebo-controlled trial of KGF for adults with ARDS did not show improvement in oxygenation metrics, ventilator-free days were fewer, and 28-day mortality was increased [99].
Children have not been included in studies of alveolar fluid clearance in patients with ARDS, and nothing is known about the rate of alveolar fluid clearance in children with ARDS as compared with adults. However, KGF is an important mediator of postnatal lung morphogenesis. Therefore, throughout childhood the lung may be “pretreated” with KGF before any insult occurs.
Surfactant
Surfactant creates variable surface tension at the air-liquid interface of the alveoli and contributes to innate immunity [100, 101]. Surfactant proteins B (SP-B) and C (SP-C) are hydrophobic and lower the surface tension of the alveolar wall liquid. The surfactant proteins A (SP-A) and D (SP-D) are collectins and contribute to innate immune responses to microbial pathogens. All four of the surfactant proteins have immunomodulatory effects and affect pulmonary fibrosis and lung remodeling [102, 103].
Adults with ARDS have low levels of SP-A, SP-B, and SP-D in bronchoalveolar lavage (BAL) fluid, and increased serum levels of these proteins in children and adults are associated with severity of lung injury [104–108]. There are lower levels and changes in the overall composition of phospholipids present in BAL fluid of patients with ARDS [109]. Reactive oxygen species from high concentrations of supplemental oxygen and activated neutrophils in the alveolar spaces of patients with ARDS may cause surfactant dysfunction [110, 111]. Finally, patients with genetic polymorphisms that result in lower levels of SP-B have a higher risk of developing ARDS, or have more severe lung injury when they become ill [112–114]. Similar findings are also seen with SP-A and SP-D with regard to development of ARDS for adults with pneumonia [115].
Several clinical trials and small case series suggested a benefit of exogenous surfactant in adults and children, but an international randomized controlled study of children with direct lung injury treated with calfactant was closed due to futility [116]. Although routine use of exogenous surfactant is not currently recommended for children with PARDS, the central role of surfactant in the pathogenesis of ARDS seems to warrant future studies [117, 118]. Secretory phospholipase A2 (sPLA2) is an enzyme with pro-inflammatory function as well as a catabolist of surfactant, and a multicenter study has been planned to investigate the role of sPLA2 in neonates and infants with lung injury [119].
Leukocytes and Inflammation
Macrophages reside in the quiescent alveolar airspace and are sentinels against pathogens [120]. Alveolar macrophages express pathogen-associated molecular pattern (PAMP) and DAMP receptors, providing early signals to the presence of pathogens and tissue injury [121]. Stimulated macrophages may also induce pyroptosis via an autocrine pathway involving the pro-inflammatory cytokine interleukin 1β (IL-1β) [122, 123]. Release of mitochondrial DNA via pyroptosis potently upregulates inflammation [25, 122]. Infiltration of activated neutrophils into the alveolar airspace is a pathologic hallmark of ARDS, but transformed alveolar and recruited macrophages, epithelial cells, and T cells also mediate the host’s innate immune inflammatory response to pathogens and lung injury [9, 26, 121, 124, 125]. In a cohort of adults with ARDS due to pneumonia, a high ratio of Treg lymphocytes to all CD4+ cells in bronchoalveolar lavage fluid collected in the first 24 h of ICU admission was associated with increased 30-day mortality [126]. Treg and T helper (Th)17 cell development and function are regulated by transforming growth factor β1 (TGF-β1), likely exerting an opposite function in immune responses, and Treg cells can be “converted” to Th17 cells [127]. In another case-control study of adults, the ratio of Th17 to Treg cells in blood collected within the first 24 h after diagnosis of ARDS was higher as compared with controls and associated with severity of ARDS and mortality [127].
Severe systemic inflammation (high levels of circulating cytokines and chemokines) activates the alveolar endothelium and circulating leukocytes resulting in “indirect” lung injury. In addition to alveolar fluid accumulation, the cell surface adhesion molecules expressed on activated alveolar endothelial cells regulate neutrophil rolling, binding, activation, and migration into the alveolar space by both para- and transcellular mechanisms [8, 128, 129].
Apoptosis
Apoptosis is a controlled, energy-dependent mechanism of programmed cell death that occurs by ligand-triggered “extrinsic” or stress-induced “intrinsic” pathways [130]. Increased alveolar epithelial cell apoptosis is associated with severity of lung injury and mortality in adults with ARDS [131, 132]. Nuclear factor kappa-B (NFκB)-dependent innate immune signaling via Toll-like receptors results in apoptotic signaling [133, 134]. Studies also suggest that Fas ligand, transforming growth factor beta (TGF-β), and lipopolysaccharide mediate inflammation, alveolar fluid clearance, and apoptosis of epithelial and endothelial cells in the lungs [92, 135–144].
Apoptosis is important for normal postnatal lung development [145–147]. Intersections between postnatal lung morphogenesis, inflammation, alveolar fluid clearance, and apoptosis may affect the outcome of children with lung injury [13, 148]. Fas has been shown to mediate apoptosis in normal lung development, and Fas/FasL may protect against hyperoxic lung injury in newborn mice [149, 150]. Fibroblast growth factor 10 (FGF-10) regulates postnatal lung morphogenesis and appears to reduce DNA damage and apoptosis of alveolar epithelial cells treated with cyclic mechanical stretch [151]. However, other animal studies suggest that mechanical ventilation during infancy may increase apoptosis in the lungs of newborns, thereby disrupting normal postnatal lung development [152]. Age-dependent mechanisms of apoptosis in the lungs of patients with ARDS remain an opportunity for future research.
Coagulation
Endothelial function, inflammation, and coagulation are inextricably linked [153, 154]. The intact glycocalyx is essential for normal intravascular anticoagulant function, and disruption of the glycocalyx has been associated with platelet activation and disseminated intravascular coagulation in sepsis [73, 155]. Activation and/or injury to the endothelium results in expression of tissue factor (TF) and von Willebrand factor (vWF) antigen, and vWF has been associated with mortality in pediatric and adult ARDS patients [156, 157]. Plasminogen activator inhibitor-1 (PAI-1) has also been associated with severity of illness and mortality in adult and pediatric patients with ARDS [8, 158, 159]. Coagulopathy and fibrinolysis are not isolated to the vascular space in the lungs of patients with ARDS, as activated protein C, thrombomodulin, and TF activity have been found in the alveolar compartment and associated with alveolar epithelial cell function [8, 158, 160].
Fibrosis and Repair
The acute pro-inflammatory response is essential to recover from direct lung injury, but prolonged inflammation in the lungs can be pathologic and lead to pulmonary fibrosis [11, 161]. Coordination of a balanced pro- and anti-inflammatory response that results in appropriate resolution of inflammation once the inciting injury has resolved requires soluble mediators, cellular immunity, and likely stem cells [162]. Catabasis is the restoration of normal organ function after an inflammatory injury [163]. Unfortunately, experimental models to investigate pulmonary fibrosis and repair have proven more challenging than ARDS models of the acute phase [164]. Since patients often present to the ICU hours to days after the initial injury has occurred, therapeutic interventions that affect the fibroproliferative phase to result in an effective “gas exchange apparatus” seems prudent [165]. Effective repair requires restoration of the air-lung and blood-lung interfaces, as well as the interstitium [166].
Coordinated activity of type II alveolar epithelial cells, macrophages, neutrophils, T cells, dendritic cells, mesenchymal stem cells, and fibroblasts is necessary for normal repair of the injured lung [86, 163, 164, 167]. Type II alveolar epithelial cells and resident mesenchymal stem cells proliferate and differentiate into type I alveolar epithelial cells, restoring the alveolar epithelium [86, 167]. The hyperplastic type II cells then undergo apoptosis to restore the normal cellular architecture of the alveolar epithelium [168]. Murine models of lung injury suggest that epithelial cell proliferation requires neutrophils and Treg cells [169, 170]. Restoration of the endothelium and glycocalyx are also necessary to clear alveolar edema and restore the normal AWL. Restoration of epithelial sodium channels (ENaC) is critical to restoring the AWL, and regulation of ENaC expression appears to be tightly linked to stem-cell-mediated reepithelialization [164, 171].
Neutrophil apoptosis is important for resolution of lung inflammation [132, 163, 172, 173]. However, studies have shown that the presence of neutrophils in the airspace is important to early fibrosis and normal repair mechanisms, suggesting that the timing of neutrophil apoptosis ideally occurs well after resolution of the inciting injury [174–176]. Resolution of inflammation and clearance of neutrophils is coordinated by Treg cells and macrophage subpopulations [121, 177–179]. Although cell-cell interactions play important roles in the resolution of inflammation, soluble mediators (IL-10, granzyme B, and lipid mediators) are also required [163, 180–182]. Studies of macrophage subpopulations suggest that they are important to both the induction and repair phases of ARDS [121, 179]. IL-10-mediated resolution of inflammation in the lung appears to require T cells and macrophages, whereas IL-4 reprograms macrophages in the absence of Treg cells [178, 183].
Remodeling the lung interstitium requires clearance of interstitial edema and remodeling of fibrin deposition [164]. Matrix metalloproteinases (MMPs) are secreted by neutrophils and appear to play an important role in the remodeling of the alveolar epithelium and extracellular matrix (ECM). [174, 184] Studies have shown that the wingless-related integration site (Wnt) signaling via β-catenin also regulates MMPs and is important early in lung injury as well as remodeling the extracellular ECM and signaling stem cells [185]. Intense research is ongoing to understand mechanisms and therapeutic targets in the ECM [162].
Stem cells derived from adult mesenchyme have limited potential to differentiate but have immunomodulatory effects. Mesenchymal stem (stromal) cells (MSCs) have been widely studied, can be expanded and cryopreserved for future use, and have an established safety for treatment of several diseases [186]. In experimental models of acute lung injury, MSCs appear to modulate inflammation, augment tissue repair, enhance pathogen clearance, and reduce severity of injury, pulmonary dysfunction, and death [186, 187]. Many of these effects occur without engraftment, but rather by paracrine effects [188]. A fixed pool of MSCs in postnatal lungs that are depleted with age may be a mechanism for age-dependent differences in outcomes of patients with ARDS.
Summary
ARDS has multiple etiologies, and the complex pathways of alveolar fluid clearance, inflammation, coagulation, apoptosis, fibrosis, and repair are regulated as a complex biological network. As definitions of ARDS in adults and children continue to evolve, knowledge of pathobiologic mechanisms will allow improvements in specificity of subtypes of the syndrome. Furthermore, overlap between regulation of postnatal lung morphogenesis and the pathobiology of ARDS suggests that the response to and outcome from a given lung injury will differ across the spectrum of age.
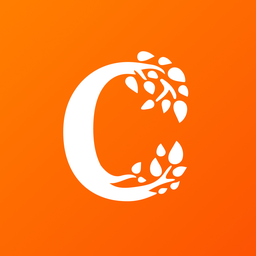
Full access? Get Clinical Tree
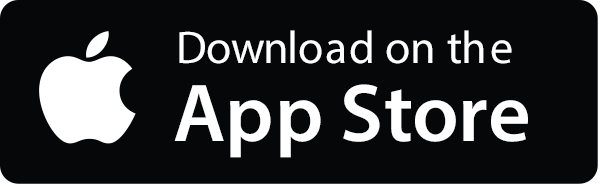
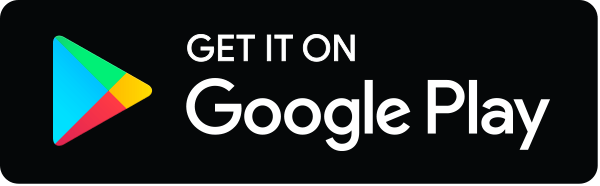