Nutritional Support and Electrolyte Management
Nutritional therapy is an integral part of the management of severely injured patients. Although malnutrition is a frequent comorbid factor in general hospitalized patients, most trauma patients are young and well nourished. Their nutritional risk is not from preexisting defects in protein stores but due to a hypermetabolic response to injury, inflammation, and sepsis. Rapid mobilization of protein from muscle supports healing, the acute-phase response, and systemic and local host barriers to infection. While tolerated for a limited time, complications during healing, sepsis, and multiple organ dysfunction syndrome (MODS) progressively drain the ability to maintain defenses and heal wounds. This chapter focuses on the role of enteral and parenteral nutritional (PN) support in reducing complications in critically injured patients with particular attention to the identification, institution, and successful management of enteral feeding.
POSTINJURY HYPERMETABOLISM
The response to the stress of injury has been classified into “ebb” and “flow” phases, which are influenced by the magnitude of injury. Changes in oxygen consumption, hyperglycemia, and increased vascular tone characterize the “ebb” phase. Epinephrine, norepinephrine, cortisol, aldosterone, and antidiuretic hormone release and exert their central and peripheral effects. Energy expenditure and body temperature are influenced through regulatory changes within the hypothalamus.1
Oxygen consumption and delivery and body temperature increase during the “flow” phase, as amino acids are mobilized into the amino acid pool from peripheral tissues for redistribution for gluconeogenesis, acute-phase protein production, immunologic proliferation, red blood cell production, and fibroblast proliferation. The magnitude of the flow phase correlates with the magnitude and severity of injury but gradually resolves unless a complication intervenes. In uncomplicated cases, diuresis of retained intracellular and extracellular water reflects resolution of this hypermetabolism as stress hormone levels drop. Appetite and gastrointestinal (GI) function return and positive nitrogen balance reappears with repletion of fat and lean body mass.
During this hypermetabolism, patients are usually immobilized and restricted by injuries and the medical therapy. Exercise is a fundamental determinant of muscle mass and immobilization of uninjured patients results in negative nitrogen balance for approximately 3–4 weeks despite adequate nutrition. Except on a very temporary basis, “positive nitrogen balance” cannot be achieved in immobilized injured patients during the first 3 weeks of hospitalization, and attempts to overfeed the negative nitrogen balance must be avoided.
THE ENDOCRINE RESPONSE TO STRESS AND INJURY
The hypercatabolism of injury, stress, and sepsis differs from that of starvation where inadequate substrate is available to meet metabolic demands; a comparison is shown in Table 60-1.2 Starvation is characterized by a decrease in metabolic rate, lethargy, a decrease in cardiac output, and a transition to ketone bodies as a major energy source. Within 18–24 hours, glycogen stores are depleted and amino acids provide the substrate for gluconeogenesis. As serum insulin levels drop, fatty acids, ketones, and glycerol become the primary substrate in tissues over 7–10 days. Ketone bodies can meet 70% of the energy requirements of the brain, normally an obligate glucose-requiring tissue. Even at full adaptation, some tissues require glucose for function. This adaptation preserves lean tissue, since amino acid demand drops and protein synthesis and catabolism decrease compared with the fed state. The respiratory quotient (RQ, the ratio of carbon dioxide production to oxygen consumed) is approximately 0.6–0.7 in starvation, indicative of utilization of fat as a primary fuel.
TABLE 60-1 Starvation versus Severe Stress Hypercatabolism
During hypermetabolism, the RQ ranges between 0.80 and 0.85. Hyperglycemia and glucose intolerance characterize this condition. Hyperglycemia is due to elevated catecholamines, which induce hepatic glycogenolysis and gluconeogenesis while inhibiting insulin secretion. Elevated cortisol stimulates glycogenolysis, muscle proteolysis, and gluconeogenesis and induces a peripheral insulin resistance to limit glucose uptake. Cortisol also inhibits gluconeogenic enzymes, potentiates hepatic effects of epinephrine and glucagon, and stimulates muscle proteolysis.3 Overall, the circulating insulin level drops and its anabolic effects are lost, resulting in increased lipolysis, fat oxidation, glucose production,4 and dependence on amino acids for glucose production.
Other hormones released with injury include glucagon and arginine vasopressin (AVP) (formerly antidiuretic hormone). Glucagon increases gluconeogenesis, glycolysis, and lipolysis, partly by reducing the insulin to glucagon ratio. AVP stimulates hepatic glycogenolysis and gluconeogenesis. Growth hormone (GH) is normally anabolic and increases glycogen deposition and protein synthesis while mobilizing fatty acids. It is inhibited following injury and during stress, as is insulin-like growth factor I (IGF-I), which is produced by the liver in response to GH. The overall effect is an imbalance in the direction of the counterregulatory hormones, a reduction in the anabolic hormones, and an accelerated loss in lean tissue. If the hypermetabolism remains unchecked, peripheral tissues become incapable of amino acid mobilization for gluconeogenesis or protein synthesis.
CHANGES IN INTERMEDIARY METABOLISM WITH STRESS AND SEPSIS
Protein Metabolism
Both protein synthesis and catabolism increase after severe trauma. Starvation, immobilization, and the hormonal and cytokine milieu factor in this response.5 Release of 3-methyl-histidine, an amino acid derived from actin and myosin metabolism in damaged and undamaged tissues, increases. Essential amino acid levels, especially branched-chain amino acids (BCAA), increase within the cell and levels of nonessential amino acids decrease, primarily through a 50% reduction in intracellular glutamine (GLN). Up to 70% of the amino acids released by skeletal muscle are alanine and GLN, although they constitute 10–15% of muscle composition. The production and metabolism of these amino acids have been studied extensively.6 During stress, skeletal muscle is capable of utilizing the BCAAs, valine, isoleucine, and leucine. While the non-BCAAs are released into the systemic amino acid pool, waste nitrogen of BCAA is disposed in two ways. In the cell, glucose is metabolized to pyruvate, which accepts a nitrogen molecule from the BCAA via transamination to create alanine. Alanine is released, cleared by the liver, deaminated, and recycled to glucose. The nitrogen is converted to urea. Additional pyruvate is converted to acetyl CoA for entry into the Krebs cycle. The transamination of nitrogen from BCAA onto alpha-ketoglutarate in the Krebs cycle produces glutamate. Transamination of a second nitrogen completes the synthesis of GLN. GLN is released and functions as a primary fuel for enterocytes and various immunologic cells (especially T-lymphocytes), and for conversion to glucose by the kidney. GLN is converted by the gut-associated lymphoid tissue (GALT) and splanchnic tissue to ammonia, ornithine, citrulline, and alanine and released into the portal circulation. Hepatic uptake clears these by-products for entry into appropriate metabolic cycles. Although serum and intracellular levels of GLN drop, overall production is increased. Waste nitrogen in the kidney is excreted as ammonia into the tubules binding hydrogen ion for elimination.
Skeletal muscle protein catabolism exceeds synthesis but there are net increases in hepatic protein production and gluconeogenesis in the liver. Synthesis of constitutive transport proteins (e.g., albumin and prealbumin) is depressed while synthesis of acute-phase proteins (e.g., C-reactive protein [CRP] and α2-acid glycoprotein) is increased. This catabolism is tolerated by well-nourished patients for a limited period, but prolonged body protein loss is associated with pulmonary, cardiovascular, metabolic, and immunologic system failure.
Glucose Metabolism
Hepatic gluconeogenesis remains elevated despite hyperglycemia. After glycogen depletion, protein provides the substrate since fat cannot be converted into glucose. Glucose infusions do not inhibit this accelerated gluconeogenesis.7 Alanine, GLN, increased lactate levels released by hypoxic tissues, and glycerol released from adipose tissue can be converted into glucose. Alanine levels increase by 40% while glycerol and lactate increase by as much as 100% in trauma patients.5 Data from burn patients8 suggest that glucose production is controlled by the liver since lowering of insulin and glucagon concentrations by somatostatin reduces hepatic glucose production despite elevated levels of substrate appropriate for gluconeogenesis.
There is evidence of less efficient glucose oxidation in both septic and trauma patients that may be secondary to intracellular derangements in control pathways caused by low pyruvate dehydrogenase levels. It is unlikely that reduced glucose oxidation is due only to insulin resistance, since glucose clearance from plasma is unrelated to glucose oxidation. This mobilization of glucose may be protective since experimental infusion of hypertonic glucose following hemorrhage reduces mortality in pigs and increases blood pressure clinically. Some effect may be due to fluid shifts due to hyperglycemia, but a positive pressor effect from increased myocardial glucose uptake has been postulated.
Fat Metabolism
Fat is the primary fuel during stress and sepsis. Increased levels of epinephrine, glucagon, cortisol, and perhaps GH enhance lipolysis following injury despite increased levels of plasma insulin. Plasma free fatty acid levels do not correlate with the degree of trauma,9 perhaps from shunting of blood from adipose tissue or drops in serum albumin since it is a transport molecule for free fatty acids. Lactic acidosis lowers free fatty acids by augmenting reesterification. The contribution of fat is evident by the depressed RQ in septic patients. As the patient recovers, the RQ increases from 0.7 of fat to 1.0 of carbohydrate, but the RQ remains depressed in septic patients.
The type of fat administered influences cellular responses. All forms of intravenous fat currently available in the United States are omega-6 fatty acids derived from vegetable oils. The omega-6 polyunsaturated fatty acid (PUFA) linoleic acid is subsequently incorporated into membranes of both immune and nonimmune cells as arachidonic acid. During stress, increased intracellular calcium releases the fatty acids by activating phospholipase A2. The products are acted upon by cyclooxygenase and lipoxygenase to produce the prostanoid PGE2, a vasodilator prostanoid, while lipoxygenase produces leukotriene B4 and 5-hydroxy-6,8,11,14-eicosatetraenoic acid (5-HETE). At high concentrations PGE2 is immunosuppressive and reduces T-cell function and migration and generation of cytotoxic cells. Leukotrienes are powerful chemoattractants that stimulate aggregation and adherence of leukocytes and natural killer cell activity. Most contemporary enteral formulas contain omega-3 fatty acids from fish and canola oil. After their subsequent release, intracellular enzymes produce prostaglandins of the 3 series (PGE3) and leukotrienes of the 5 series, which are less immunosuppressive and not as proinflammatory as the omega-6 products. Clinically, these cell membrane increases are measurable within a few days of administration.10
Current Issues
Nutritional support is but one very important aspect in the overall management of the trauma patient. Most trauma patients are well nourished, although geriatric patients and younger patients with histories of substance abuse may have varying degrees of malnutrition. Debridement, drainage, resection of infected tissue, stabilization of fractures, adequate resuscitation, etc., are the priorities in early management, because early control will influence the subsequent metabolic response. If sources of inflammatory mediators are not controlled, nutrition support will not preserve catabolic lean tissue. With control, nutritional support can maintain host defenses, preserve lean body mass, improve patient outcome, and, if administered early via the GI tract, reduce infections.
Aggressive nutrition support in critically injured patients is an invasive form of therapy with risks and benefits that cannot be approached casually. In elective surgical patients undergoing general surgical procedures, the risks of therapy can, in some circumstances, outweigh benefits.11 However, in patients who are malnourished or at risk of becoming so, the benefits outweigh the risks if nutritional support is carefully instituted and monitored. There are significant clinical data demonstrating that after gaining hemodynamic stability, severely injured patients who are fed early via the GI tract have a reduced risk of septic complications and, in some circumstances, MODS.12–17 The availability of effective tools to quantitate the magnitude of injury and likelihood of subsequent septic complication allows surgeons to identify patients for whom enteral access is warranted at the time of initial celiotomy.
Not all patients can be fed enterally, particularly if enteral access is not obtained at the initial laparotomy. In these situations, intravenous nutrition should be instituted on the fifth day, since there is no evidence that early intravenous nutrition significantly improves clinical outcome. However, when it is clear that the GI tract is not functioning or will not function, PN is indicated.
ENTERAL VERSUS PARENTERAL FEEDING: THE CLINICAL ARGUMENT
The preponderance of evidence demonstrates benefits with enteral feeding and the elective use of PN early after trauma is associated with increased infections.38 The most compelling data exist in randomized, prospective clinical studies12–18 of victims of blunt and/or penetrating trauma. After the first clinical data of improved outcome with enteral feeding shown in pediatric burn patients,19 subsequent trauma studies compared various enteral products with either no feeding or intravenous feeding. Most showed reductions in intra-abdominal abscess and pneumonia in the enterally fed group. Moore et al. randomized patients with an abdominal trauma index (ATI) between 15 and 40 to early feeding with a defined-formula diet or no early feeding and showed a 3-fold reduction in sepsis (primarily intra-abdominal abscess) with enteral feeding.13 Subsequently, they showed that enteral feeding significantly reduced pneumonia compared with PN14 consistent with prior20 work showing a lower septic rate in patients receiving most of their nutrition enterally.
The Memphis group showed that enteral feeding significantly reduced pneumonia (11.8% vs. PN 31%), intra-abdominal abscess (1.9% vs. PN 13.3%), and line sepsis (1.9% vs. PN 13.3%).12 Severity of injury influenced the results since the reduced infection rate occurred in patients with an Injury Severity Score (ISS) >20 and an ATI >24 or both, where PN increased infectious rates by 6.3, 7.3, or 11 times, respectively.
A few studies produced conflicting data. One group found similar rates of infection in trauma patients receiving intravenous feeding or enteral nutrition with no difference in pneumonia or intra-abdominal infection.21 However, there were nearly twice as many head injuries, three times as many severe thoracic injuries, and three times as many pelvic fractures in the enterally fed patients. The increased incidence of severe chest injuries in the enteral group made the diagnosis of pneumonia suspect, since pneumonia was diagnosed using the standard criteria of fever, leukocytosis, and a new or changing infiltrate on chest x-ray, which overdiagnoses pneumonia in more than two thirds of cases.22
Another study randomized blunt trauma patients requiring intensive care unit (ICU) care to either early (<24 hours) or late (>72 hours) enteral feeding.23 Total infectious complications—ranging from pneumonia to an eye infection—were significantly greater in the early fed group but there was a very high dropout rate, and the early fed patients had more severe pulmonary injury, as measured by the Pao2/FiO2 ratio. Eleven of 19 patients in the early group had a Pao2/FiO2 ratio <150, while only 4 of 19 patients in the late group had severe pulmonary dysfunction. Pneumonia occurred twice as often in the early fed patients, but results were confounded by the increased chest trauma.
DETERMINING INDICATORS FOR ENTERAL (POSTPYLORIC) ACCESS DURING CELIOTOMY FOR TRAUMA
The ATI (Table 60-2) can be rapidly tabulated at the operating table to determine the need for direct small bowel access.24 Patients with an ATI ≥25 appear to benefit from early enteral nutrition once they are hemodynamically stable. Patients with insignificant intra-abdominal injuries will benefit from early feeding if they sustain significant extra-abdominal injuries such as a severe pulmonary contusion; multiple rib fractures; a closed head injury with a Glasgow Coma Scale (GCS) score ≤8; a spinal injury; a major soft tissue injury requiring repeated irrigation, debridement, and skin grafts; or multiple extremity fractures necessitating later surgery. In addition, patients with multiple chronic diseases or patients who will require prolonged ventilator support or have delayed resumption of oral intake are appropriate candidates. Most patients with these criteria have an ISS >20 and benefit from the early enteral feeding (Fig. 60-1). Severe intra-abdominal injuries to the colon, pancreas, liver, or duodenum increase the risk of septic complications, but enteral access is usually unnecessary with relatively minor, isolated injuries to these organs.
TABLE 60-2 Calculated Risk of Sepsis by the Abdominal Trauma Index
FIGURE 60-1 Protocol for nutrition support at the University of Wisconsin-Madison and the Elvis Presley Trauma Center at the University of Tennessee, Memphis.
Patients with Closed Head Injury
There is little evidence that early nutrition affects outcome after severe head injuries. We wait until GI tract function returns and gastroparesis resolves (generally within 3 days) before instituting intragastric feeding. Prolonged gastroparesis (>6 days) unresponsive to gastric motility agents requires that a tube be advanced into the distal duodenum and ideally beyond the ligament of Treitz. Intravenous nutrition should start if this is not possible.
This approach stems from a critical review of the head injury studies. Rapp et al.25 randomized 38 patients to PN or delayed enteral feeding (>1 week) once gastroparesis resolved. Initial work suggested improved GCS score at 3 months with early PN but no difference after this point. This result was not confirmed in a follow-up study.26 Grahm et al.27 randomized head-injured patients to enteral feeding via nasojejunal tube placed fluoroscopically within 36 hours of injury or via a gastrostomy tube once gastric atony resolved, usually within 5 days. Fewer bacterial infections and fewer ICU days occurred after early enteral feeding. However, bronchitis was the major diagnostic category separating the groups, rather than established infections such as pneumonia or abscess. Borzotta et al.28 randomized patients with severe head injuries to early enteral feeding via nasojejunal tube or delayed enteral feeding and demonstrated no reduction in infections and a slight, perhaps clinically insignificant improvement in neurologic outcome with early feeding. A Memphis study29 found no difference between an early placement of a tube beyond the ligament of Treitz and intragastric feeding, with no improvement in GCS or infectious complications.
The failure of enteral nutrition to reduce infections in head-injured patients appears contradictory to multiple trauma results, but the groups differ significantly. Prolonged intubation and pneumonia may be unavoidable after severe closed head injury, since patients with such injuries often remain intubated for weeks, while many multiple trauma patients require support only for several days.
Burn Patients
Early enteral feeding in burn patients is well established. Alexander et al.19 randomized severely burned children to either a standard enteral diet or a protein-supplemented diet. Patients receiving the high-protein diet had a higher survival rate and a lower sepsis rate but received significantly less PN than control patients. Herndon et al.30 randomized 39 patients with 50% or greater total body burn to early IV or enteral feeding and measured significant decreases in natural killer cell activity and the T-helper/suppressor ratio with PN. Since higher survival rates occurred with enteral feedings, they concluded that PN should be used only with total enteral failure.
Intragastric feeding should be started within 12 hours of burn, since this approach reduces gastroparesis. Gastroparesis occurs in 45% of patients with intragastric feeding delayed for 18 hours, but it occurs in less than 5% of patients if feeding is instituted within 8 hours of burn. Laboratory evidence suggests a blunting of the hypermetabolic response after burn by reducing levels of cortisol and catecholamines.
INFLUENCE OF THE TYPE OF ENTERAL FEEDING ON INFECTIOUS COMPLICATIONS
Specialty “Immune-Enhancing” Diets
The concept that some nutrients became “conditionally essential” during stress and sepsis (e.g., GLN) or that other substrates such as omega-6 fatty acids could potentially aggravate inflammation through production of proinflammatory products led to the creation of a family of immune-enhancing diets (IEDs). These IEDs contain various combinations of GLN, arginine, omega-3 fatty aids, beta-carotene, and nucleotides. Liquid diets cannot be supplemented with GLN as a free amino acid because of its propensity to degrade into the toxic products pyroglutamate and ammonia after heat sterilization. Free GLN is found only in dry, powdered diets, which require reformulation prior to administration. These diets can be administered via transgastric jejunostomies (TGJs), large-bore (12–18 Fr) jejunostomies, and 7-Fr needle catheter jejunostomies (NCJs), but they tend to clog 5-Fr NCJs.
The rationale for these specific nutrient supplementations has been partly discussed above. Although skeletal muscle production of GLN increases during stress and sepsis, serum and intracellular levels decrease. GLN is a substrate for various immunologic cells and for enterocytes in the unfed state.6 Arginine promotes proliferating T cells after mitogen or cytokine stimulation in vitro and increases natural killer cell cytotoxicity, specific cytolytic T-cell activity, and macrophage tumor cytotoxicity. It also exerts a positive effect on wound healing; stimulates pituitary GH, prolactin, insulin, and IGF-I, and acts as a precursor for nitric oxide, nitrites, and nitrates as well as the growth substances putrescine, spermine, and spermidine, which may be involved in GI tract integrity. Omega-3 PUFAs from fish or canola oil can replace omega-6 PUFAs, such as linoleic acid, found mainly in vegetable oils. Omega-6 fatty acids inhibit killer cell activity, antibody formation, and cell-mediated immune response due to the inhibitory nature of their metabolic end products.10 Animal studies have shown that omega-3 supplementation reduces mortality after burn injury, reduces bacterial translocation compared with other lipids, promotes cell-mediated immunity, increases resistance to infection, reduces inflammation, and reduces mortality following bacterial peritonitis. The administration of RNA via nucleotides improves survival to a septic challenge with Candida albicans, and malnourished animals provided RNA during refeeding from a malnourished state had more rapid improvement in immune function.31 Nucleotide deprivation suppresses helper T-cell and interleukin-2 (IL-2) production, largely due to uracil, since adenine does not prevent this immunosuppression. Clinically, various combinations of these nutrients have been made available in specialty nutrient diets. The majority of clinical studies carried out in critically ill patients, general surgical patient populations, trauma patients, and burn patients suggest an additional benefit with the IEDs over unsupplemented diets.16,17,32,33
Gottschlich et al.34 randomized 50 pediatric and adult patients with burns over 10–89% of their body surface area to diets including a modular feeding supplemented with arginine, cysteine, histidine, and omega-3 fatty acids. A significant reduction in wound infection, length of stay per percent body burn, and total number of infectious complications occurred in this group, with a trend (P = .06) toward reduced pneumonia. More inhalation injuries in the control group as well as more fat and less carbohydrates in the control formula were confounding variables.
Patients with an ATI between 18 and 40 randomized to an IED or a chemically defined diet generated significant increases in total lymphocyte count and T-lymphocyte and T-helper cell numbers as well as fewer intra-abdominal abscesses and less multiple organ failure with the supplemented diet.17 Since a higher nitrogen content in the IED was a confounding variable, a subsequent study randomized patients with an ATI >25 or an ISS >20 to an IED or an isonitrogenous, isocaloric diet.16 Unfed cohorts, eligible for the trial entry but without enteral access, were also prospectively followed. The IED resulted in fewer major septic complications, fewer therapeutic antibiotics, and a shorter hospital stay than in the unfed group or those receiving the control diet. The incidence of intra-abdominal abscess, pneumonia, bacteremia, major wound infections, or any major complication was highest in the unfed population. Complications and antibiotic use with the control diet were between the unfed and IED groups. Administration of a GLN-supplemented enteral diet has also been shown to reduce pneumonia, bacteremia, and sepsis.35 Potential negative effects may also occur with these diets. One study noted an increase in respiratory failure with an IED.36 An increased incidence of respiratory failure in the treatment group at baseline limited the ability to implicate the diet, however.
We institute an IED in patients with an ATI >25 or an ISS >20 (Fig. 60-1) and convert to a standard high-protein enteral diet once the patients are less vulnerable to septic complications, usually within 7–10 days. Patients with less severe injuries receive a high-protein diet.
POSSIBLE DELETERIOUS EFFECTS OF IMMUNONUTRITION IN THE CRITICALLY ILL
Recently published Canadian practice guidelines for nutrition support in mechanically ventilated critically ill patients recommended that arginine-containing diets (oral immunonutrition that contains arginine supplementation) should not be used in critically ill ICU patients.37 The conclusion was reached despite significantly reduced hospital stays and trends toward reduced ventilator days and ICU days noted with these diets in trials. This meta-analysis based these recommendations on three studies. One trial of trauma, surgical, and medical ICU patients randomized patients to a diet enriched in arginine, omega-3 fatty acids, and nucleotides.33 Patients tolerating more than 821 mL per day benefited clinically in a post hoc analysis, but septic patients randomized to the arginine-containing diet had increased mortality, although overall mortality was not affected. A second randomized but unpublished prospective study conducted in 1996 randomized critically ill patients to a specialized diet containing moderate amounts of arginine and omega-3 fatty acids. Mortality increased with this supplemented diet in elderly male patients admitted with sepsis and pneumonia. However, significantly more patients with pneumonia were randomized to the specialty diet due to a randomization breakdown. Most patients who died either received less than 3 days of feeding or did not receive the specialty diet long after completion of the study period. A third study38 randomized septic patients requiring more than 4 days of nutrition support and a “higher level of care” to PN or an omega-3 fatty acid/arginine-supplemented enteral diet. Increased mortality occurred in this group but most deaths occurred in patients septic with pneumonia similar to unpublished trial noted above.
Although there are significant reservations regarding the design and implementation of these three trials, septic patients—particularly if septic with pneumonia—may have a poorer outcome with immunonutrition for unknown reasons, although increased nitric oxide production during sepsis, but not stress, has been proposed.39 Two trials noted no increase in mortality with sepsis using similar diets.40,41 With the clinical evidence of improvement in trauma patients, there is no evidence to suggest this population has increased risks with administration of these specialty diets and guidelines have recommended their use.42
POTENTIAL REASONS FOR IMPROVED OUTCOME WITH ENTERAL FEEDING
The unfed GI tract has altered function and architecture. Decreases in villus height occur in unfed human patients, but to a lesser extent than in rat models of starvation or intravenous feeding, where 40–50% of villus height is lost in the proximal intestine. Changes occur quickly in the GI tract following shock and stress, with rapid decreases in enzymes and motility and temporary increases in permeability. The reduced motility (i.e., ileus) occurs in the stomach and colon, allowing absorption of nutrients delivered into the small intestine.
Shock, trauma, and sepsis increase gut permeability, which increases bacterial translocation to mesenteric lymph nodes in animal models and under certain clinical conditions, such as bowel obstruction, inflammatory bowel disease, and shock. This does not appear to be clinically relevant following trauma.43 Increased permeability in trauma patients is unrelated to the magnitude of trauma but does correlate with the IL-6 and acute-phase protein response of severely injured patients. The level of permeability returns to baseline within a week.
The well-fed intestine is a metabolically and immunologically active organ that passively and actively maintains a bacterial barrier through peristalsis, IgA, mucin, and mucosal cell integrity. Experimentally, these systems fail in starvation, shock, and sepsis. Approximately 75% of the body’s immunoglobulin-producing cells line the GI and respiratory tracts to produce 70–80% of the immunoglobulin as secretory IgA (SIgA), which is transported across the mucosa.44 The GALT is affected by PN, producing decreases in GALT mass and reductions in intestinal and respiratory IgA. Normally, the IgA coating the surface of the epithelium binds and neutralizes viruses, bacteria, and other pathogenic antigens by trapping them in the mucin layer. As a result, these infective agents cannot attach to the mucosa, a prerequisite to invasive infection. Experimentally, PN-fed animals lose established antibacterial and antiviral immunity.44 Under conditions of stress, ischemia, and injury, Alverdy and Chang demonstrated that intraluminal bacteria respond to this hostile environment by upregulation of virulence genes and expressing adhesins that render them more virulent in vivo.45
Intraperitoneal protection can also be explained by similar mechanisms. Enteral feeding significantly improves the survival of malnourished or well-nourished animals with septic peritonitis by increasing intraperitoneal tumor necrosis factor (TNF) response, resulting in a lower systemic cytokine response and bacteremia compared with animals fed intravenously. A differential cytokine response has also been confirmed in patients. Normal volunteers administered PN demonstrate an increase in production of TNF compared with enterally fed patients following intravenous injection of endotoxin.46 Since TNF has been considered one of the precipitating stimuli for the subsequent proinflammatory cytokine response, this may be clinically relevant.
Exposure of the systemic circulation to bacterial products has been postulated to be a driving force in the development of MODS.47 Enteral stimulation maintains a normal gut cytokine response with high levels of IL-4 and IL-10.44 These cytokines upregulate IgA production from mucosal protection and down-regulate adhesion molecule expression on the vascular endothelium. Parenteral feeding reduces these cytokines, leading to an upregulation of vascular adhesion molecules and accumulation of neutrophils within the intestine and priming of these neutrophils to subsequent ischemic events.82 Ischemic events within the gut have been previously related to neutrophil priming as an initial step in MODS development.48 These experimental data are consistent with the reduction in MODS in trauma patients fed enterally.17
THE NUTRIENT PRESCRIPTION: ISSUES COMMON TO ENTERAL OR PARENTERAL FEEDING
There are a number of issues common to both enteral and parenteral feedings related to the amount and type of nutrition administered to trauma patients. In the past, calculation of nutrient needs used standard equations, such as the Harris–Benedict equation, multiplied by correction factors. Indirect calorimetry has shown that these correction factors for stress and activity tend to overestimate nutrient needs. Overfeeding leads to increased oxygen consumption, increased CO2 production, hepatic lipogenesis, potential immune suppression secondary to hyperglycemia, and other negative effects without reducing weight loss or lean tissue catabolism.
ESTIMATING NUTRITIONAL NEEDS
The most common calculation used to determine basal energy expenditure (BEE) is the Harris–Benedict formula, defined as follows:
where W is the weight in kilograms, H is the height in centimeters, and A is the age in years. These calculated values have been increased by a stress factor (ranging from 1.25 to 2) and an activity factor to account for the increases in metabolic rate by injury and stress.
Indirect calorimetry measures metabolic needs using expired gas analysis to determine overall resting energy expenditure (REE). Carbon dioxide production (co2) and oxygen consumption (
o2) are used to calculate the RQ. When
o2 and
co2 are applied to the Weir equation, REE can be determined. There is an RQ characteristic for each fuel utilized: for example, fat is 0.7, glucose is 1.0, and protein is 0.8. Since fat deposition with overfeeding has an RQ value of approximately 8, an RQ value greater than 1.0 generally means that lipogenesis and overfeeding are occurring. In studies of patients in surgical ICUs, metabolic cart measurements were generally within 5–10% of the energy requirements calculated by the Harris–Benedict equation; addition of stress factors led to unnecessary administration of nutrients.49
There are drawbacks to indirect calorimetry. Since o2 is an integral part of the measurement, there is loss of accuracy as the FiO2 increases because of the errors between the inspired and expired oxygen consumptions. With an FiO2 of 0.8, a 1% error in measurement of the inspired or expired
o2 leads to 100% error in
co2 calculation. Since air leaks are interpreted as increases in oxygen consumption, critically ill patients requiring a high FiO2 and high positive end-expiratory pressure are most likely to have inaccurate measurements. In addition, these calculations are labor-intensive and results are most reliable when protocols defined by trained technicians are used. Indirect calorimetry measurements only reflect caloric needs over a 20- to 30-minute period. When the patients are turned, suctioned, or receiving physical therapy, those measurements may not represent total energy expenditure. For these reasons, many practitioners increase the REE by 10–15% to calculate total energy expenditure. Because of the expenses necessary to avoid the pitfalls of indirect calorimetry, its routine use is not recommended. However, data obtained from sites proficient in the technique have provided valuable guidelines to avoid overfeeding, especially in cases of obesity, amputation, or quadriplegia, where traditional calculations are not reliable.
Current recommendations for caloric support range between 25 and 30 total kcal/kg per day. While some studies have recommended other values, if 30 kcal/kg is provided, 90% of patients will reach their energy requirement with overfeeding in approximately 15–20% of patients.49 To avoid complications of overfeeding (Fig. 60-2), we administer approximately 25–30 total kcal/kg per day to multiply injured patients initially and decrease this to 22–25 kcal/kg as they improve clinically, with diuresis, ventilator weaning, and discontinuation of antibiotics. Presumed body weight is obtained from family members or the patient, when possible, to avoid overfeeding based on weights increased by edema. As diuresis occurs, weight is rechecked with appropriate adjustments in total caloric load. In general, we withhold nutrition in patients requiring high rates of catecholamine infusion, since there is no evidence that administered glucose or protein (or amino acids) slows lean body tissue mobilization or the rate of gluconeogenesis.
FIGURE 60-2 Complications of overfeeding. (Reproduced with permission from Frankel WL, Evans NJ, Rombeau JL. Scientific rationale and clinical application of parenteral nutrition in critically ill patients. In: Rombeau JL, Caldwell MD, eds. Clinical Nutrition. Vol. 2. Parenteral Nutrition. 2nd ed. Philadelphia: Saunders; 1993:597, © Elsevier.)
Stress induces an increase in both glucose utilization and gluconeogenesis with significant recycling of glucose from lactate (the Cori cycle) and alanine. Lactic acid is produced in the periphery when pyruvate cannot enter the Krebs cycle. As NADPH is converted to NADP, hydrogen ion is transferred to pyruvate creating lactate, which is then released, transported back to the liver, and converted to glucose. Alanine is formed by transamination of pyruvate during BCAA metabolism in skeletal muscle and released for conversion back to pyruvate and glucose by the liver. Hyperglycemia occurs as hepatic gluconeogenesis increases from 2.0 to 2.5 mg/(kg min) under the normal condition to 4–5 mg/(kg min) in the stressed or septic patient. Given these conditions, high levels of infused glucose aggravate hyperglycemia causing glucosuria, hepatic fatty deposition, increased CO2 production (rarely a significant clinical problem in young trauma victims), and potentially an increased infection rate. Since the maximal rate of glucose oxidation is 5 mg/(kg min) or approximately 7.2 g/kg per day, cumulative glucose administration including enteral and parenteral products as well as intravenous fluids should not exceed these levels. In a 70-kg adult, this is 500 g of glucose at maximum, which is almost completely met by 2 L of a 25% dextrose PN solution. Ideally, blood sugars should be maintained below 180 mg/dL. With insulin, it is usually possible to keep blood sugars below 180 mg/dL except in trauma patients with insulin-dependent diabetes mellitus or those with steroid or high catecholamine needs.50
ENERGY REQUIREMENTS AND FEEDING RECOMMENDATIONS IN THE MORBIDLY OBESE
Morbid obesity is becoming more and more prevalent in the trauma and surgical ICUs. The World Health Organization now classifies obese patients into three categories: Obese Class I are patients with BMIs of 30–34.9, Obese Class II are patients with BMIs of 35–39.9, and Obese Class III are patients with BMIs ≥40. Current feeding recommendations for morbidly obese trauma or surgical ICU patients are for Class I or Class II obesity, 22 total kcal/kg ideal body weight and 2 g of protein/kg ideal body weight. For patients who are in the Obese Class III category, recommendations are for slightly higher calories of 22–25 total kcal/kg of ideal body weight and 2–2.5 g protein/kg of body weight.51 This is based on observations that nitrogen retention increases as caloric intake increases until caloric intake approaches 50–60% of total energy expenditure. Additional energy further improves nitrogen retention but less efficiently. Although weight loss occurs, this is primarily due to fat loss.
In a 2-week study of enteral feeding52 this population received 1.5–1.9 g/kg ideal body weight per day of protein with either 19 kcal/kg actual body weight per day (30 total kcal/kg ideal body weight per day) or 11 total kcal/kg actual body weight per day (hypocaloric group: 22 total kcal/kg ideal body weight per day). Prealbumin increases were similar in the two groups. Both had negative nitrogen balance due to catabolism, but the hypocaloric group had a shorter ICU stay, fewer antibiotic days, and a trend toward reduced ventilator days (P <.09).
Hypocaloric, parenteral high-protein feeding of postoperative obese patients with 2.1 g of protein and 20 total/kcal ideal body weight resulted in 1.7 kg per week of weight loss, net protein anabolism (improved serum protein concentration and positive nitrogen balance), and successful wound healing.53 Two other randomized double-blind studies of obese patients compared hypocaloric feeding with higher-calorie regimens. In the first, both groups received 2 g/kg ideal body weight per day but calories were administered at 100% or 50% of the measured energy expenditure.54 Nitrogen balances were similar. In the second, hypocaloric or higher-calorie parenteral regimens (14 ± 3 total kcal/kg actual weight per day vs. 22 ± 5 total kcal/kg actual weight per day) were given for up to 14 days with both groups receiving 2 g/kg ideal body weight of protein. Nitrogen balances were similar, but glucose control was improved and less exogenous insulin was necessary with the hypocaloric regimen.55
FAT REQUIREMENTS
Free fatty acids are the primary energy source utilized during stress and sepsis. Lipolysis and mobilization of free fatty acids is enhanced by beta-adrenergic stimulation of lipases and hormone-sensitive tissues as fatty acid oxidation increases. The administration of glucose as calculated above can provide approximately 50–60% of total caloric requirements; the balance of nonprotein calories (NCPs; 20–30% of total calories) can be met by fat at a dose of 1 g/kg per day. Infusion rate for lipids should not exceed 0.11 g/kg/hr. In patients in whom ventilator weaning is an important issue, administration of fat as total calories up to 50% may be beneficial in selected patients. Increased CO2 production is rarely a cause of weaning problems in trauma patients except, perhaps, in geriatric patients following flail chest injuries or those with prolonged recovery. Trauma patients with moderate to severe chronic obstructive pulmonary disease (COPD) who have trouble weaning from the ventilator may benefit from a diet higher in fat. If overfeeding is suspected, reduction in total calories administered is the most appropriate intervention. Higher doses of fat may also be useful in hyperglycemic patients as a result of diabetes or corticosteroid administration. This may cause hyperlipidemia, cholestasis, increased risk of infection, and perhaps immunosuppression. All lipid emulsions available in the United States are vegetable oils, which provide mainly omega-6 long-chain PUFAs; these may increase production of immunosuppressive prostaglandins and leukotrienes. Currently, structured lipids containing both omega-6 and medium-chain triglycerides as their primary fatty acid side chains are being tested as are intravenous fats with omega-3 fatty acids. Additional immunosuppression may come from blockade of the reticuloendothelial system by lipid particles. Omega-3 fatty acids from canola or fish oil are found in most enteral products; these can be metabolized to the less immunosuppressive 3 or 5 series of prostaglandins. Omega-3 PUFAs may increase the production of lipid peroxidases and inhibit platelet aggregation, but these concerns have not appeared to be of clinical significance.
Several investigators have determined the maximum hydrolysis rate for intravenous lipids by LPL in normal adults to be approximately 0.12 g/kg/hr (2.9 g/kg per day); above this infusion rate emulsion triglyceride particles begin to accumulate. Intravenous lipid emulsion in the critically ill adult below this infusion rate is recommended in order to avoid elevated triglyceride concentrations and other metabolic complications. Doses of intravenous lipids should be limited to the provision of essential fatty acids (e.g., 250 mL of 20% lipid emulsion once or twice weekly) when triglyceride concentrations rise above 400 mg/dL. Withholding intravenous lipids must be considered when triglyceride concentrations are greater than 500 mg/dL or in the presence of lipemic serum.
PROTEIN REQUIREMENTS
Skeletal muscle loses considerable mass as amino acids are redistributed for healing wounds, acute-phase proteins, and substrate for lymphoid tissue and the GI tract. Overall, nitrogen is lost through ureagenesis by the liver and ammonia synthesis by the kidney. Immobilization aggravates the loss. Total protein catabolism exceeds synthesis, which leads to loss of function and potential morbidity and mortality. Unfortunately, this hypercatabolism and lean tissue loss is not blunted by the administration of exogenous calories or amino acids.
In general, the recommended dose of amino acid protein for stressed or septic patients without renal dysfunction is 1.5–2 g/kg per day. Although blood urea nitrogen (BUN) may increase to approximately 40 mg/dL, these levels cause no metabolic complications and BUN generally stabilizes at that level. If urea levels climb to greater than 100 mg/dL, protein administration must be reduced. If creatinine levels are low, that is, no evidence of acute renal failure (ARF), and elevated BUN appears due to excessive protein (or amino acid) administration, protein should be decreased to 1.3–1.5 g/kg per day. As the stress phase resolves, 1–1.5 g/kg per day of protein meets nutrient requirements in most patients. In burn patients, administration of 2–2.5 g/kg per day is desirable due to excessive urinary losses and the inability to accurately assess wound losses of nitrogen.
Patients who develop ARF secondary to injury/hypotension still require high doses of protein for wound repair; however, it is practical to provide only 0.8–1.0 g/kg per day of protein until a decision on initiation of dialysis is made. Once dialysis has been initiated, the type and frequency will dictate how much the protein dose can be liberalized. Generally, 1.2–1.5 g/kg per day can be administered to a patient receiving hemodialysis three times a week (assuming that the hemodialysis is effective). Patients who cannot tolerate hemodialysis secondary to hypotension may receive continuous arterial venous hemodialysis (CAVHD) or continuous venovenous hemodialysis (CVVHD). These methods of dialysis provide the critical care practitioner volume to administer PN with nonconcentrated formulas, and protein can usually be administered as high as 2.5 g/kg per day if indicated.
THE FORMULA
Calorie-to-Nitrogen Ratio
Caloric density for nutrients is as follows: 1 g protein equals 4 kcal, 1 g of hydrated glucose equals 3.4 kcal, and 1 g of fat (as an enteral form) equals 9.1 kcal, but 1 g of lipid emulsion contains 10 kcal/g because of glycerol contained within the emulsion. In patients who are not critically ill or septic but at risk of developing starvation-induced malnutrition if not fed (e.g., small bowel obstruction, prolonged intestinal paresis, multiple small bowel fistulas), a calorie:nitrogen ratio between 130:1 and 160:1 is appropriate, and protein should be administered at a dose of 1–1.5 g/kg per day. In patients who are stressed or septic, however, the calorie:nitrogen ratio should drop to 80:1 to 120:1. The goal in the stressed or septic patient is approximately 30 kcal/kg total with 1.5–2 g/kg per day of protein. In burn patients, protein is administered with approximately the same low calorie:nitrogen ratio, providing 35–40 kcal/kg per day total and 2–2.5 g/kg per day of protein. Refer to Figure 60-3 for sample calculations of a PN formulation involving a 70-kg trauma patient.
Example: 70-kg man sustaining severe multiple long-bone fractures
Estimated kcal: 30 kcal/kg per day × kcal/day
Protein: 1.5 g/kg per day × 70 kg = 105 g/day
For 2-in-1 (dextrose/amino acid) formulation:
Using a 70% dextrose (D70W) stock solution for compounding:
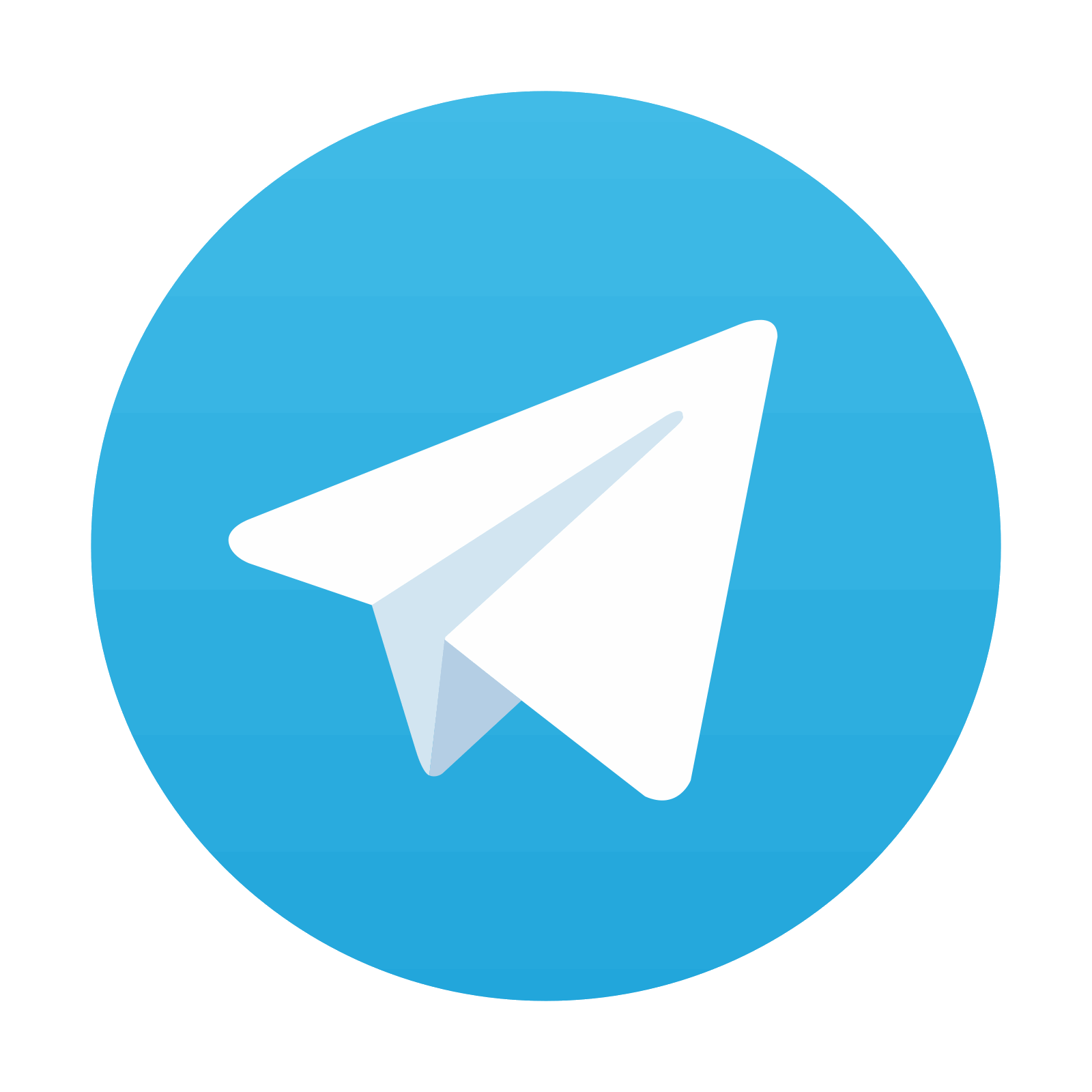
Stay updated, free articles. Join our Telegram channel
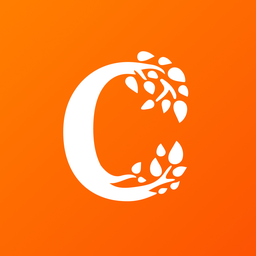
Full access? Get Clinical Tree
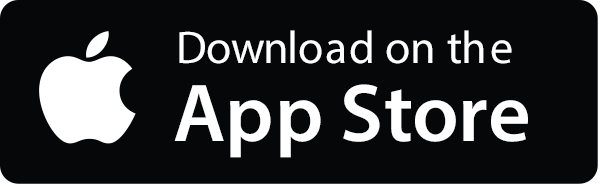
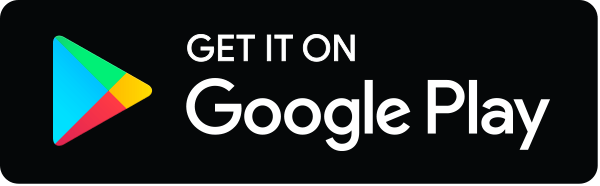