Abstract
Nutrition is a vital consideration in children with critical heart disease for several reasons: (1) nutritional deficiency may cause heart disease; (2) congenital heart disease (CHD) may cause malnutrition and poor growth, which can delay or complicate corrective surgery; (3) and congenital heart surgery may be associated with complications that lead to life-threatening metabolic and nutritional derangements. Children with CHD frequently are malnourished before surgical intervention; however, emerging evidence suggests safety and tolerance of preoperative enteral nutrition. The extent of undernutrition has a significant impact on the timing of surgical interventions, as well as the perioperative outcome. Preoperative enteral nutrition is feasible in many children. Postoperative nutritional support should be instituted early and aggressively. Enteral feedings should be employed whenever possible, and parenteral nutrition should be reserved for those unable to be fed enterally. Given the significant probability of postoperative dysphagia in many children with CHD, particularly those undergoing Norwood procedures, a multidisciplinary swallowing evaluation before oral feeding may be required. Gastroesophageal reflux, protein-losing enteropathy, and persistent chylothorax are significant postoperative complications with important nutritional complications in children with CHD. A staged therapeutic approach consisting of dietary changes, medical interventions, and then invasive interventions, if needed, is successful in mitigating the nutritional complications of each of these conditions in a majority of cases.
Key Words
Malnutrition, energy requirements, growth, enteral nutrition, parenteral nutrition
Nutrition is a vital consideration in children with critical heart disease for several reasons: (1) nutritional deficiency may cause heart disease; (2) congenital heart disease (CHD) may cause malnutrition and poor growth, which can delay or complicate corrective surgery ; and (3) congenital heart surgery may be associated with complications that lead to life-threatening metabolic and nutritional derangements.
Nutritional Deficiency as a Cause of Heart Disease
Malnutrition (Protein-Energy Deficiency)
Protein-energy malnutrition (PEM) is the inappropriate loss of body cell mass secondary to reduced intake or inadequate use of substrate. Children, especially neonates, are particularly prone to developing PEM because they have minimal metabolic reserve to combat illness. PEM may arise from reduced intake or inadequate absorption leading to caloric deprivation or may be a result of a hypermetabolic inflammatory process that triggers inadequate use of protein substrate while nonprotein caloric intake remains relatively unaffected. The prevalence of PEM in hospitalized children was previously reported as 36% to 54%, but in 2010 the estimates were 1.3% to 28%. This lower prevalence may be related to inconsistencies in documentation and coding of malnutrition, which have changed in recent years. Other pediatric studies in intensive care units continue to find deficiencies in meeting caloric and micronutrient needs. Children with malnutrition continue to have worse outcomes compared with nourished children. The distinction between starvation and hypermetabolism is important and will affect the choice of nutritional therapy to prevent the detrimental complications of underfeeding or overfeeding. Because PEM contributes greatly to morbidity in heart disease, it is important to understand this entity.
Metabolic Adaptations in Starvation.
Glucose is the principal energy substrate in the body and vital for the central nervous system and red blood cells, so the body adapts to preserve glucose homeostasis during starvation ( Fig. 26.1 ). Hepatic glycogenolysis provides the first defense for glucose homeostasis during starvation. However, the hepatic glycogen supply is depleted after 24 hours in adults and sooner in infants. A fall in serum glucose concentration results in a release of the counterregulatory hormones epinephrine, cortisol, and glucagon. These hormones stimulate proteolysis to provide amino acids for gluconeogenesis. Increased counterregulatory hormones combined with decreased levels of insulin stimulate lipolysis and accelerate the production of ketone bodies and fatty acids, which serve as alternative fuel sources in the brain, heart, and muscle. After several days the brain adapts to the use of ketones, and the rate of muscle proteolysis declines.

Specific Micronutrient Deficiency Syndromes and Heart Disease
Iron Deficiency.
Iron deficiency is a common and potentially life-threatening problem in children with cyanotic CHD. It is associated with hypercyanotic attacks (“tet spells”), cerebral thromboses, metabolic acidosis, and infection especially in the younger child. Because the adequacy of tissue oxygen delivery is dependent on the hemoglobin concentration, and thus indirectly on iron stores, maintenance of iron sufficiency is paramount.
The iron-deficient cyanotic patient may exhibit an elevated red blood cell count with relatively normal hemoglobin concentration. Another clue to the presence of iron deficiency in this population comes from the fact that the hematocrit is usually greater than three times the hemoglobin level, in contrast to the usual 3 : 1 ratio in the iron-sufficient patient. The mean corpuscular volume may also be low, reflecting the presence of a hypochromic microcytic anemia. The child with critical heart disease and iron deficiency should receive iron therapy. The supplemental iron administration is usually required for over a month until the hematocrit increases. Serial hematocrits during this period are essential, because some patients will experience rapid increases in hematocrit after iron therapy and develop polycythemia (hematocrit >60%). Polycythemia increases blood viscosity and also increases the risk of cerebral thromboses.
l-Carnitine Deficiency and Fatty Acid Oxidation Defects.
Recurrent exacerbations of systemic carnitine deficiency or a fatty acid oxidation defect are heralded by vomiting, diarrhea, and mental status changes associated with nonketotic hypoglycemia, hyperammonemia, elevated liver transaminases, lactic acidosis, and coagulopathy. The cardiac manifestations include right or biventricular hypertrophy and cardiomegaly with decreased contractility. This presentation is reminiscent of Reye syndrome with encephalopathy, shock, and weakness.
l -Carnitine is an essential cofactor in fatty acid oxidation and energy production for myocardial contraction. This dependence on fatty acid metabolism as a source of energy is especially acute during periods of fasting, when the glucose supply is inadequate.
Primary carnitine deficiency results in low plasma and tissue carnitine levels and generally presents with varying combinations of cardiomyopathy, skeletal muscle weakness, and encephalopathy. Laboratory examination usually reveals hypoketotic hypoglycemia. The specific carnitine enzyme defects associated with critical heart disease are given in Table 26.1 .
Enzyme Defects Associated With Carnitine Deficiency | Features |
---|---|
Carnitine-acylcarnitine translocase deficiency | Life-threatening episodes in the neonatal period Cardiac arrhythmia Generalized weakness Hyperammonemia Variable hypoglycemia |
Carnitine palmitoyltransferase II deficiency (hepatocardiomuscular form) | Infants or children Cardiomegaly Cardiac arrhythmia Liver disease Encephalopathy Fasting hypoketotic hypoglycemia Mild myopathy Sudden death |
Primary systemic carnitine deficiency (carnitine membrane transporter deficiency) (defect on chromosome 5q) | Dilated cardiomyopathy ECG: peaked T waves Endomyocardial biopsy: massive lipid storage and low carnitine concentration Poor response to inotropes and diuretics Dramatic response to carnitine supplementation Acute encephalopathy Hypoketotic hypoglycemia Hepatomegaly with liver steatosis Myopathy |
Secondary carnitine deficiencies are the result of excessive carnitine losses or inadequate carnitine intake or production. Inadequate carnitine production in the liver may arise in cirrhosis, in malnutrition, or after valproate therapy. Carnitine intake may be deficient with chronic parenteral nutrition (PN) or certain vegetarian diets. Excess carnitine losses may occur with diuresis, diarrhea, or hemodialysis.
Fatty acid oxidation defects include very long chain acyl-coenzyme A (CoA) dehydrogenase (VLCAD) deficiency, long-chain acyl-CoA, long-chain L-3-hydroxyacyl-CoA dehydrogenase (LCHAD) deficiency, or medium-chain acyl-CoA dehydrogenase (MCAD) deficiency, and glutaric acidemia type II. Patients with these enzymopathies typically have low plasma and tissue carnitine levels and an associated cardiomyopathy. The specific diagnosis is made based on the serum acylcarnitine profile and elevated urinary dicarboxylic acids. Table 26.2 lists the specific clinical findings in fatty acid oxidation defects.
CLINICAL FINDINGS | LABORATORY FINDINGS | |||||||||||
---|---|---|---|---|---|---|---|---|---|---|---|---|
Defects | CM | AR | SD | RM | HM | EC | ↓ Glu | ↓ Ket | Met Acid | ↓ Car | ↑ LFTs | ↑ NH 3 |
VLCAD | ✓ | ✓ | ✓ | ✓ | ±✓ | ✓ | ✓ | ✓ | ✓ | ✓ | ✓ | ✓ |
MCAD | ✓ | ✓ | ✓ | ✓ | ✓ | ✓ | ✓ | |||||
LCHAD | ✓ | ✓ | ✓ | ✓ | ||||||||
child | ||||||||||||
GA II | ✓ | ✓ | ✓ | ✓ | ✓ | ✓ |
Glucose supplementation during periods of stress is the most important therapy for patients with any of the fatty acid oxidation defects. However, some patients have been successfully treated with carnitine supplementation. An inverse correlation between survival and plasma carnitine levels has been demonstrated in cardiomyopathic patients. l -Carnitine supplementation in these circumstances normalizes mitochondrial function and myocardial performance. Critically ill children with carnitine deficiencies should receive adequate dietary carnitine supplementation and have their serum glucose levels monitored frequently to avoid potentially catastrophic hypoglycemia. Carnitine supplementation has been successfully used in children with cardiomyopathy.
Medium-chain triglyceride (MCT) administration may alleviate the cardiac manifestations of secondary carnitine deficiency. MCTs are transported across the mitochondrial membrane independently of carnitine and are mainly oxidized. Consequently, MCTs provide a greater energy substrate than long-chain triglycerides (LCTs) and may minimize the consequences of a secondary carnitine deficiency.
Thiamine Deficiency.
Beriberi is caused by a dietary deficiency of thiamine. Thiamine pyrophosphate, vitamin B 1 , acts as a cofactor for pyruvate dehydrogenase and other enzymes involved in the pentose and tricarboxylic acid pathways needed to metabolize pyruvate and lactate in the Krebs cycle. In developed nations thiamine deficiency occurs in alcoholics and in selective diets. Childhood beriberi is seen in patients receiving unsupplemented PN and in infants breast-fed by thiamine-deficient mothers. Thiamine deficiency may occur in critically ill children; 12.5% of children receiving care in a pediatric intensive care unit (PICU) for more than 2 weeks and 18% of children admitted for surgical correction of complex congenital heart defects were thiamine deficient. Clinicians should have a low threshold for suspecting and treating thiamine deficiency in critically ill children given the severe neurologic consequences. Long-term treatment of heart failure with furosemide may also predispose patients to thiamine deficiency.
There are three forms of beriberi heart disease. Shoshin beriberi is acute fulminant myocardial depression, on the verge of acute cardiovascular collapse. These patients are dyspneic, cyanotic, and display restlessness with an increasing metabolic acidosis. Physical examination reveals severe tachycardia, massive cardiomegaly, jugular venous distention, and hepatomegaly in the absence of peripheral edema. Although thiamine supplementation rapidly restores peripheral vascular resistance, improvement in contractility is often delayed. Acute infantile beriberi presents between the ages of 1 and 4 months in children breast-fed by thiamine-deficient mothers. Infants are pale, irritable, edematous, and often hoarse. Trivial infections can precipitate acute, life-threatening Shoshin beriberi. In the chronic form, wet beriberi, patients appear diaphoretic and edematous with sinus tachycardia, a widened pulse pressure, and a diminished arteriovenous extraction of oxygen. Skeletal muscle blood flow is increased at the expense of cerebral, hepatic, and renal perfusion.
Vitamin D and Calcium Deficiency.
Vitamin D deficiency may both contribute to and be a result of pediatric cardiac dysfunction. Vitamin D is a pleotropic hormone that is not only important to bone health but also impacts cardiac function and immunomodulation. Vitamin D deficiency is associated with cardiomyopathy, organ dysfunction, and prolonged PICU stay.
Many infants with CHD have vitamin D deficiency. Although infants are often deficient preoperatively, Vitamin D levels drop intraoperatively during initiation of cardiac bypass and with the administration of catecholamines. It is still unknown if this is a modifiable risk factor that affects outcome. In the outpatient setting, vitamin D supplementation has been associated with improved cardiac function.
Hypocalcemia, as a manifestation of hypoparathyroidism, is seen in the 22q11 deletion syndromes, DiGeorge syndrome or velocardiofacial syndrome. Ten percent to 20% of these will present with hypocalcemia in the first 3 months of life; 10% of these babies will present with hypocalcemic seizures.
Other factors that affect calcium, vitamin D, and bone mineralization may be more specific to the surgery itself. Following the Fontan operation, children and young adults are more prone to skeletal muscle deficits and vitamin D deficiency compared with their peers. Additionally, decreased bone density has been seen in children who have undergone Fontan palliation compared with other forms of CHD, despite normal vitamin D levels. Hyperparathyroidism has also been described in children following Fontan palliation.
Refeeding Syndrome With Hypophosphatemia.
Children with PEM are at risk for developing refeeding syndrome, which manifests as hypophosphatemia and other electrolyte derangements if caloric intake is advanced too quickly. Patients may have normal serum phosphorus concentrations, but their total body phosphorus is depleted. During nutritional intervention, anabolic processes increase cellular uptake of serum phosphate, usually between the second and fifth day, but this can occur as late as 7 to 10 days after initiating feeding. If serum phosphate levels fall below 1 mg/dL, encephalopathy, diaphragmatic failure, dysrhythmias, acute renal failure, or hepatocellular injury may result. Refeeding syndrome can be prevented by gradually increasing caloric supplementation, although aggressive nutritional support and vigilant phosphate monitoring and replenishment may be just as effective.
Multifactorial Nutritional Syndromes in Children With Critical Heart Disease
Overview
Undernutrition and growth retardation are common complications of CHD. The magnitude of growth failure can be astounding and continues to be a significant problem. Over 70% of children with cardiac disease are below the 50th percentile for height and weight, 50% are below the 16th percentile for height and weight, and over 35% are below the 3rd percentile for both height and weight. Prolonged nutritional deficits and growth failure related to congestive heart failure may result in heightened surgical risks, particularly in infants with single-ventricle defects. The etiology of undernutrition seen in children with CHD is multifactorial. Contributing factors include age at surgery, the increased energy expenditure of frequent infections and congestive heart failure, as well as inadequate intake due to dysphagia and frequent feeding interruptions, feeding intolerance, and intestinal malabsorption.
Hypermetabolism in Uncorrected Congenital Heart Disease
A meta-analysis comparing 65 infants with CHD and 124 healthy controls found that total daily energy expenditure in infants with CHD is 35% more than that of healthy controls. Presumably the elevated catecholamine levels increase oxygen consumption significantly. This increase in basal metabolic rate (BMR) reduces the calories that usually supply growth during infancy and childhood. Even if a normal caloric intake could be ensured, this would be insufficient to replenish the deficit. Often a caloric intake of 150% of normal values for age is necessary to support normal growth in this population.
Insulin and Glucose Homeostasis in Congenital Heart Disease
In children with CHD, alterations in insulin secretion and glucose homeostasis can occur. Children with symptomatic ventricular septal defects have normal fasting glucose levels and glucose tolerance test results but lower insulin levels and surprisingly higher rates of insulin secretion, as demonstrated by higher plasma concentrations of C-peptide. The higher rates of insulin secretion seen in children with CHD are a consequence of high levels of sympathetic activity, resulting in increased glucose release. Conversely, children with cyanotic heart disease have lower fasting glucose levels, normal glucose tolerance, and insulin levels with higher rates of insulin secretion. If the cyanotic infant has inadequate glucose stores, hypoglycemia may be the net result of the high insulin secretion rate. Conversely, increases in pulmonary circulation increase insulin clearance , which may minimize the risk of hypoglycemia in CHD. Following cardiac surgery, infants are likely to experience transient hyperglycemia, which is often associated with intraoperative glucocorticoid administration.
Growth Hormone Insensitivity
Children with CHD and malnutrition may be insensitive to growth hormone (GH), which may partially explain growth failure in this population. Normally GH affects metabolism in diverse ways. Its effect on protein metabolism is anabolic and leads to positive nitrogen balance. Conversely, GH has catabolic effects on fat metabolism by increasing lipolysis. The resultant increase in free fatty acids accounts for the ketogenic effect of GH. GH has an antiinsulin effect and raises serum glucose levels by increasing hepatic glucose output and decreasing glucose uptake into muscle.
GH promotes growth through its effects on cartilage and protein metabolism, which require the interaction between GH and somatomedins, such as insulin-like growth factors (IGFs) 1 and 2. GH stimulates local IGF secretion in various tissues, including liver, cartilage, and heart. Local and circulating IGFs stimulate growth. Insulin-like growth factor–binding protein 3 (IGFBP-3), also synthesized under GH control, is the most important carrier of circulating IGF-1. The GH:IGF-1:IGFBP-3 axis plays an important role in the preservation of anabolism following stress.
This axis is altered in critical heart disease. Malnourished children with CHD have elevated levels of circulating GH but markedly decreased levels of IGF-1 and IGFBP-3, suggesting GH insensitivity. Children undergoing cardiac surgery for complex CHD exhibit this GH resistance preoperatively. In the initial postoperative week the discrepancy between elevated GH levels and depressed IGF-1 and IGFBP-3 worsens. These GH:IGF-1: IGFBP-3 abnormalities resolve within 6 months after corrective surgery. GH also regulates ventricular size and myocyte contractility. Both Turner syndrome and Noonan syndrome are associated with congenital cardiac anomalies and short stature and frequently require GH replacement.
Heart Failure
Heart failure may result in poor appetite, tachypnea, vomiting, or dysphagia. Others have emphasized the importance of anorexia and early satiety in the development of this malnutrition state. When heart failure is mild, the hungry infant overfeeds, resulting in fluid overload and impaired cardiorespiratory function. Increased patient fatigability during oral feeding then limits feeding volumes, resulting in underfeeding, as well as prolonged feeding times. Persistent vomiting, thought to be a manifestation of left-to-right intracardiac shunts, which resolves following surgical correction, may be an additional contributor to inadequate intake. Underweight children with CHD have inadequate diets compared with their normal-weight cohorts. With nutritional counseling these children demonstrate a 10% weight gain from baseline over a 6-month period.
Intestinal Malabsorption and Nutrient Loss (Protein-Losing Enteropathy, Chylothorax)
Children with CHD may accrue nutritional deficits through intestinal malabsorption or leakage of nutrients into the intestinal lumen or the pleural space. In some settings the nutrient leakage follows the malabsorption syndrome. Intestinal malabsorption may result from heart failure and is thought to be a form of intestinal lymphangiectasia. As right heart failure increases, lymphatic drainage from the gut is impeded, and a functional lymphatic obstruction develops. Consequently, intestinal absorption and digestion of protein and fats are altered. Carbohydrate absorption is generally normal. Fat malabsorption may occur in some patients with symptomatic congestive heart failure regardless of the underlying cardiac anomaly, but not if the heart failure has been treated effectively with diuretics.
Absorption of protein can be impaired in children with heart disease, particularly in patients with a single ventricle who have undergone the Fontan operation. This protein-losing enteropathy (PLE) is thought to be due, in part, to increased systemic venous and mesenteric lymphatic pressure resulting in the leakage of protein in the lumen of the gut (see Chapter 15 on splanchnic function for a full discussion of PLE, including dietary and therapeutic options).
Chylothorax , a well-recognized complication of thoracic procedures, has an incidence of 0.25% to 1.9% after surgery for CHD. These chylothoraces are thought to be the result of intraoperative damage to the thoracic duct and accessory lymphatic channels. Systemic venous hypertension can also result in chylothorax formation by impeding lymphatic duct emptying. Chylothorax should be suspected whenever a pleural effusion develops after a thoracic procedure, including both median sternotomies and thoracotomies. Chylothorax following thoracotomy was noted to present later in the postoperative period when compared with median sternotomy. Additional risk factors for chylothorax after congenital heart surgery include the Fontan procedure itself and central line insertion in either the subclavian or internal jugular vein. Chylothorax is a significant complication because large chylous effusions result in immune, nutritional, and cardiovascular derangements secondary to the loss of T lymphocytes, chylomicrons, and electrolyte-containing fluid.
If the patient has been receiving enteral nutrition, including long-chain fatty acids, chyle has a creamy appearance because it contains chylomicrons and LCTs. The diagnosis of a chylothorax is confirmed by the presence of a pleural fluid triglyceride level of more than 1.2 mmol/L and a total cell number of greater than 1000 cells/µL, consisting predominantly of lymphocytes. However, in the typical postoperative case of chylothorax, the child has been fasting. Therefore the chylous effusion may appear serosanguinous without an elevated triglyceride content. Although an elevated lymphocyte count in the pleural effusion may suggest the diagnosis of chylothorax, the laboratory analysis is often nondiagnostic. The diagnosis is then made retrospectively after therapy directed at chylothorax results in resolution of the effusion. The initial management consists of tube thoracostomy for large effusions and dietary modification ( Fig. 26.2 ).

Nutritional management of chylothorax traditionally includes a regimen that is very low in LCTs and high in MCTs and contains sufficient protein and electrolytes to replace nutrient losses in chyle. The use of MCT-enriched diets is based on the understanding that enterocytes directly absorb medium-chain fatty acids into portal circulation, reducing lymphatic flow and enabling healing of the damaged lymphatic vessels. Commercially prepared infant formulas containing at least 80% of fat as MCT oil can be used to meet the nutrient needs of infants with chylothorax. Application of a MCT diet alone was effective in resolving the chylothorax in 71% of patients in one study. The use of fat-free human milk may be an important dietary approach to consider in infants with chylothorax due to its immunologic qualities. Through the process of cold centrifugation, human milk can be separated into a solidified-fat top layer and a lower fat-free liquid portion. Because removal of the fat portion causes the human milk to become deficient in energy, essential fatty acids, and fat-soluble vitamins, the fat-free human milk must be supplemented with MCT oil, vitamins, carbohydrate modulars, and/or high-MCT infant formula. If the use of fat-free human milk is prolonged (greater than 2 to 4 weeks), supplementation with 2% to 4% of total energy in the form of essential fatty acids may be necessary to prevent essential fatty acid deficiency. This is illustrated in a recent report demonstrating no difference in the resolution of chylothoraces with fat-modified breast milk versus MCT infant formula; however, the fat-modified breast milk group experienced a decline in mean weight and height. If clinical symptoms of chylothorax persist (no decrease in chylous chest tube output) on a low LCT/high MCT diet, complete enteric rest and PN may be required. This approach is successful in a majority of cases. Clinical improvement is defined as a decrease in effusion drainage to less than 10 mL/kg/d. More than a third of patients receiving fat-free formulas will experience resolution of their chylothoraces within 2 weeks. Nutritional management of chylothoraces is typically continued for up to 6 weeks.
Symptomatic venous thrombosis is commonly associated with chylothorax and may contribute to its severity and duration. Of 1396 children who underwent cardiac surgery, 54 developed chylothorax and 28 (52%) had venous thromboembolism confirmed by ultrasonography. Indeed, elevated central venous pressures are associated with more refractory chylothoraces. These chylothoraces resolve slowly because of the time it takes to reach a sufficiently high intralymphatic pressure to cause lymphatic rupture. Such patients require a careful hemodynamic evaluation to identify the cause of the systemic venous hypertension. Potential causes in the surgical patient include the aforementioned systemic venous thrombosis, which requires anticoagulation, or pulmonary artery stenosis, which may require stent placement or surgical plasty. Recently balloon angioplasty for the treatment of left innominate vein obstruction in the face of chylothorax led to improvement if not full resolution of the chylothorax. Chylothorax associated with postoperative pulmonary hypertension can be treated with a trial of nitric oxide (NO), which decreases right ventricular afterload and associated tricuspid regurgitation and/or augments forward flow through the pulmonary circulation.
Somatostatin and its synthetic analogue, octreotide, have been used successfully to treat chylothorax. There is a subset of patients who seem to respond to octreotide; they have lower chest tube output, and a higher proportion are patients with single-ventricle anatomy. By acting directly on splanchnic vascular receptors, octreotide reduces chylomicron synthesis and transport into the lymphatic duct, thus decreasing lymphatic flow rate and limiting triglyceride loss into the pleural fluid. Octreotide has been used both as a continuous infusion and in divided subcutaneous doses. It is initiated at 10 mcg/kg/d in three divided subcutaneous doses and increased in a stepwise fashion of 5 to 10 mcg/kg/d every 3 days until chyle output decreases to minimal levels for 3 consecutive days. At this point the octreotide is weaned over the next 3 to 6 days. The dose of octreotide required to achieve total suppression of chylous effusion was reported as 20 to 40 mcg/kg/d.
Although as many as nearly 80% of patients respond to dietary and medical management to resolve their chylothoraces, the remainder do not and require surgical interventions. Such surgical techniques include pleurodesis with talc/tetracycline or fibrin glue, video-assisted thoracoscopic surgical (VATS) identification and clipping of the site of duct leakage or thoracic duct ligation, or pleuroperitoneal shunting. The operations are preceded by consumption of a meal of cream mixed with Sudan black 1 hour before the procedure to identify the leak site. In four of six adults with a chylothorax the site was successfully identified using a VATS technique, then sealed with fibrin glue and sutures or clips. The remaining two underwent pleurodesis with fibrin glue. All of these chylothoraces resolved completely by the fifth postoperative day. Despite these impressive results, a right-sided thoracic duct ligation, done using either a VATS or open approach, remains the standard procedure for a refractory chylothorax. When this fails, consideration should be given to a left-sided approach with left periaortic mass ligation due to the anatomic variation in pediatric CHD patients, especially those with dextrocardia.
In two small series of children with persistent chylothorax, implantation of double-valved pleuroperitoneal shunts resulted in successful resolution of their symptoms, without complications. These shunts are removed an average of 1 to 2 months post placement. An advantage of a pleuroperitoneal shunt is to minimize the loss of chyle by facilitating its absorption by the peritoneum and allowing the formation of new lymphatic thoracic channels. The use of these shunts is limited by high right atrial pressure (>25 mm Hg) or by inferior vena cava obstruction.
Nutritional Assessment
Anthropometrics
Growth is the primary outcome measure of nutritional status in children and should be monitored at regular intervals from infancy through adolescence and at every preventive, acute, or chronic care encounter. In children less than 36 months of age, growth parameters should include weight-for-age, length-for-age, head circumference-for-age, and weight-for-length. In children 2 to 20 years of age, growth parameters include weight-for-age, standing height-for-age, and body mass index (BMI)-for-age.
The best indicator of growth failure is the change in growth rate over time. Longitudinal growth is preserved during acute short-term nutritional deprivation. Therefore a low weight-for-length percentile in children less than 2 years of age and a low BMI percentile in children greater than 2 years of age suggest an acute energy imbalance, whereas a low length-for-age or height-for-age percentile indicates long-standing deficits. Many children presenting with cardiac disease weigh significantly less than normal for age in the face of appropriate height.
Anthropometric measurements provide the best estimates of muscle and fat content of the body in the clinical setting. In the absence of edema, measuring triceps and subscapular skinfold thickness assesses adipose tissue but is most accurate when serial measurements are taken by the same trained clinician. Skeletal muscle mass can be estimated by measurements of the arm muscle circumference and is useful in assessing the depletion of lean body mass. Mid-upper arm circumference (MUAC) can be used an independent marker in determining malnutrition in children 6 to 59 months of age. MUAC measurements are particularly important in those whose weights alone are unreliable due to fluid status, such as those with lower extremity edema or ascites or those receiving steroids. MUAC has been indicated as a more sensitive prognostic indicator for mortality than weight-for-length or BMI in malnourished pediatric patients. The Academy of Nutrition and Dietetics and the American Society for Parenteral and Enteral Nutrition (ASPEN) have developed standardized guidelines for identifying and documenting pediatric malnutrition ( Tables 26.3 and 26.4 ).
Mild Malnutrition | Moderate Malnutrition | Severe Malnutrition | |
---|---|---|---|
Weight-for-length z-score | −1 to −1.9 | −2 to −2.9 | ≥ −3 |
BMI for age z-score | −1 to −1.9 | −2 to −2.9 | ≥ −3 |
Length/height for age z-score | No data | No data | ≥ −3 |
Mid-upper arm circumference z-score | ≥ −1 to −1.9 | ≥ −2 to −2.9 | ≥ −3 |
Mild Malnutrition | Moderate Malnutrition | Severe Malnutrition | |
---|---|---|---|
Weight gain velocity (<2 years) | <75% of the norm for expected weight gain | <50% of the norm for expected weight gain | <25% of the norm for expected weight gain |
Weight loss (2-20 years of age) | 5% usual body weight | 7.5% usual body weight | 10% usual body weight |
Deceleration in weight-for-length or BMI z-score | Decline of 1 z-score | Decline of 2 z-scores | Decline of 3 z-scores |
Inadequate nutrient intake | 51%-75% estimated energy/protein needs | 26%-50% of estimated energy/protein needs | ≤25% estimated energy/protein needs |
Biochemical Measurements
Nitrogen balance reflects the equilibrium between protein intake and losses. Stress produces nitrogen losses, driven by the catabolic actions of cortisol and epinephrine. Skeletal muscle breakdown provides substrate for gluconeogenesis and also releases nonessential amino acids that are excreted in the urine as urea. All patients with PEM have a negative nitrogen balance. The magnitude of the negative balance is useful in determining the process leading to the patient’s malnourished state. In hypermetabolism, tissue is being catabolized for gluconeogenesis and wound repair. Acute negative nitrogen balance is the rule. Chronic starvation leads to a modestly negative nitrogen balance. Drainage of proteinaceous fluids from other body cavities will further increase nitrogen loss.
Although serum protein concentrations (particularly albumin and transferrin) have been used as biochemical markers of nutritional status, these tests are neither sensitive nor specific. Hypermetabolism is associated with negative nitrogen balance, but hypoalbuminemia requires prolonged hypermetabolism because of the long half-life of albumin (20 days) ( Table 26.5 ). Hypoalbuminemia in the face of a hypermetabolic state is associated with increased risk of death. The shorter half-life proteins such as retinol-binding protein and prealbumin correlate better with acute changes in critically ill patients. However, prealbumin levels can be affected by several factors. Lower levels may be seen in liver disease, or they may be falsely elevated in renal failure and steroid use. Visceral proteins such as albumin and prealbumin do not accurately reflect nutritional status and response to nutritional intervention during inflammation. During periods of inflammation the liver reprioritizes synthesis of acute-phase proteins such as C-reactive protein (CRP) over transport proteins such as prealbumin. Therefore levels of serum prealbumin and CRP are inversely related. For the postoperative infant, decreases in serum CRP level to less than 2 mg/dL have been associated with the return of anabolic metabolism and are followed by increases in serum prealbumin levels.
Plasma Protein | t 1/2 |
---|---|
Transferrin | 8 d |
Albumin | 20 d |
Prealbumin | 2 d |
Retinol-binding protein | 10 h |
Predictive Methods of Energy Expenditure
An infant’s caloric requirement depends on his or her BMR, the rate of growth, and activity. Because adipose tissue is not as metabolically active as other tissue, an undernourished infant with little or no subcutaneous fat has a higher than expected level of metabolism relative to body weight. The caloric requirements of recovering undernourished children are also increased because of active reparative anabolic processes. Fever significantly increases total energy requirements. Each degree Celsius elevation in body temperature increases caloric needs by 12%.
The nutritional needs of patients in intensive care rarely conform to tabular norms because these patients are not in a basal state. The term resting energy expenditure (REE) is used for adults when they are supine and quiet or asleep and have not eaten for at least 3 hours. REE is a function of gender, age, height, and weight. However, studies of critically ill children on mechanical ventilation in whom energy expenditures were measured by indirect calorimetry reported wide differences between measured and predicted values, even after stress factors were applied.
Consistent with the ASPEN guidelines, indirect calorimetry to measure REE provides the best estimate of energy requirements in critically ill pediatric patients. Several studies comparing indirect calorimetry versus predictive equations in estimating energy requirements in the critically ill child following cardiac surgery found that predictive equations are unreliable and do not take into account the unpredictable metabolic changes that occur in these patients. Because the metabolic response is difficult to estimate and changes throughout the acute and recovery phases, individualized nutrition prescriptions using indirect calorimetry every second day should be the new standard in this population.
If indirect calorimetry is unavailable, basing REE on standard equations without additional stress factors during the acute phase response can help prevent overfeeding and its potential consequences. The end of the acute/catabolic stress response may be estimated through serial monitoring of CRP levels (CRP <2 mg/dL suggests a return to anabolic state). The most commonly used equations for critically ill patients are the World Health Organization (WHO) equations to calculate REE and the Schofield equations to calculate BMR ( Tables 26.6 and 26.7 ).
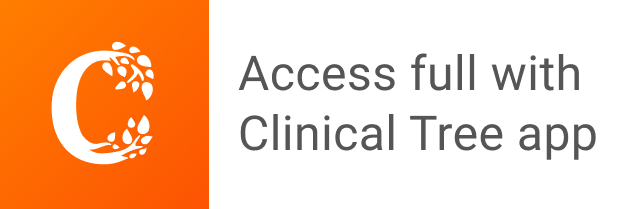