The proper collection and interpretation of hemodynamic waveforms are important components of cardiac catheterization, yet they are often overshadowed by the more glamorous aspects of cardiology, such as angiography and coronary or structural intervention. Accurate intracardiac pressure measurements provide invaluable physiological information in both normal and disease states; poorly gathered or erroneously interpreted waveforms may lead to an incorrect diagnosis or a wrong clinical decision. It is imperative that competent cardiologists understand normal and abnormal hemodynamic waveforms and can troubleshoot problems. It is also important to recognize common artifacts and understand potential pitfalls and certain unique circumstances that may occur during the collection and interpretation of hemodynamic data.
GENERATION OF PRESSURE WAVEFORMS
The goal of a hemodynamic study is to accurately reproduce and analyze the changes in pressure that occur within a cardiac chamber during the cardiac cycle. These rapidly occurring events represent mechanical forces and require conversion to an electrical signal to be transmitted and subsequently translated into an interpretable graphic format. The pressure transducer is the essential component that translates mechanical forces to electrical signals. The transducer may be located at the tip of the catheter (micromanometer) within the chamber or, more commonly, the pressure transducer is outside the body, and a pressure waveform is transmitted from the catheter tip to the transducer through long connecting tubing containing a column of saline fluid. These transducers consist of a diaphragm or membrane attached to a strain gauge-Wheatstone bridge arrangement. When a fluid wave strikes the diaphragm, an electrical current is generated with a magnitude dependent on the strength of the force that deflects the membrane. The output current is amplified and displayed as pressure versus time.
During the cardiac cycle, changes in pressure occur rapidly, corresponding with various physiological events. The force wave created by these events generates a spectrum of wave frequencies. Consider, for example, the different events that occur within the aorta throughout a single cardiac cycle. Beginning with left ventricular ejection, the aortic valve opens, causing pressure to steadily rise in the aorta, rapidly reaching a peak, and then falling quickly following peak ejection. Closure of the aortic valve represents another event, marking the end of systole and is associated with a slight rise in pressure during the pressure decay phase and then followed by continued pressure decay during ventricular diastole until the next ventricular contraction. All these events occur rapidly and are associated with varying wave frequencies. When the force wave strikes the transducer, it should precisely reproduce these events. The full range of frequencies needed to reproduce these force waves requires a sensing membrane capable of a rapid frequency response (0–20 cycles/s in the human heart). However, the physical properties of a membrane capable of such a wide frequency response might resonate, creating artifacts. This phenomenon is similar to the sound that a bell makes, continuing to oscillate after initially struck. Resonation artifact appears on the waveform as excessive “noise,” but reverberations can also lead to harmonic amplification of the waveform overestimating systolic pressure and underestimating diastolic pressure ( Fig. 2.1 ). Therefore hemodynamic measurement systems need to provide some level of “damping” to reduce resonation artifacts. Damping is a method of eliminating the oscillation; it may be done by the introduction of friction to reduce the oscillation of the sensing membrane, or it may be accomplished electrically by damping algorithms. However, note that damping reduces the frequency response and thus may result in loss of information. Overdamping results in the loss of rapid high-frequency events (e.g., the dicrotic notch on the aortic waveform), causing underestimation of the systolic pressure and overestimation of the diastolic pressure. The ideal pressure tracing has the proper balance of frequency response and damping.

CALIBRATION, BALANCING, AND ZEROING
Previous generations of transducers required calibration against a mercury manometer; this is no longer needed with the factory-calibrated, disposable, fluid-filled transducers in clinical use today. Table-mounted transducers do require balancing or “zeroing,” which refers to the establishment of a reference point for subsequent pressure measurements. The reference or “zero” position should be determined before any measurements are made. By convention, it is defined at the patient’s midchest in the anteroposterior dimension at the level of the sternal angle of Louis (fourth intercostal space) ( Fig. 2.2 ). This site is an estimation of the location of the right atrium and is also known as the phlebostatic axis . A table-mounted transducer is placed at this level, and the stopcock is opened to air (atmospheric pressure) and set to zero by the hemodynamic system. The system is now ready for pressure measurements.

The tradition of using the midaxillary line as the zero position has been called into question. Because of the influence of hydrostatic pressure in the supine position, and the use of fluid-filled transducers, some physicians believe that setting the zero position as the upper border of the left ventricle is more accurate. The difference between this location and the conventional location provides the greatest accuracy in diastolic pressure measurements. However, most routine laboratories find this approach impractical because it requires the use of echocardiography to determine the precise location; it is more applicable to research investigations. A major advantage of the midchest position is that it has been shown to correlate with the position of the left atrium by magnetic resonance imaging studies, regardless of the patient’s age, sex, body habitus, or presence of chronic lung disease. Frequently, busy catheterization laboratories might position the transducer at the same level for all patients, or from a measured, fixed distance from either the table or from the top of the patient’s chest, without taking into consideration the variations in patient position or body habitus. This practice will lead to marked inaccuracies, particularly in patients who are unable to lie flat or who are at the extremes of body weight. A transducer placed above the true zero position will produce a pressure lower than the actual pressure; a transducer placed below the true zero position will result in a pressure measurement higher than the actual pressure. These small pressure changes caused by improper zeroing will be more impactful in the lower, diastolic heart pressures and may lead to significant errors in diagnosis and, perhaps, inappropriate therapy.
Transducer drift refers to either the loss of calibration or the loss of balance after initially setting the zero level. This is not uncommon. Many patients have been started on pressors for hypotension or have been falsely diagnosed with mitral stenosis because of inaccurate transducer balancing, improper zero positioning, or transducer drifting. Careful attention to this aspect is important for proper interpretation. When in doubt, if the findings do not make sense with the clinical scenario, or if the result is needed for an important decision, recheck the zero.
NORMAL PHYSIOLOGY AND WAVEFORM CHARACTERISTICS
Interpretation of pressure waveforms requires a consistent and systematic approach ( Box 2.1 ). After confirming the zero level, the scale of the recording is noted, and a recording sweep speed is determined. Establishing standard protocols is helpful to ensure that all necessary information is collected in a systematic format (see Chapter 1 ). Careful scrutiny of the waveform ensures a high-fidelity recording without overdamping or underdamping. Each pressure event should be timed with the electrocardiogram (ECG). Finally, the operator should review the tracings for the presence of common artifacts that might lead to misinterpretation.
- 1.
Establish the zero level and balance transducer
- 2.
Confirm the scale of the recording
- 3.
Collect hemodynamics in a systematic method using established protocols
- 4.
Critically assess the pressure waveforms for proper fidelity
- 5.
Carefully time pressure events with the electrocardiogram
- 6.
Review the tracings for common artifacts
The various events occurring during the cardiac cycle create characteristic waveform appearances. Fig. 2.3 demonstrates the three basic waveforms (atrial, ventricular, and arterial) and their relationships to the key electrical and physiological events during the normal cardiac cycle. Each waveform will be described in detail in the following sections.

Right Atrial Waveform
The normal right atrial pressure is 2 to 6 mm Hg and is characterized by a and v waves and x and y descents ( Fig. 2.4 ). The a wave represents the pressure rise within the right atrium due to atrial contraction and follows the P wave on the ECG by about 80 ms. The x descent represents the pressure decay following the a wave and reflects both atrial relaxation and the sudden downward motion of the atrioventricular (AV) junction that occurs because of ventricular systole. An a wave is usually absent in atrial fibrillation, but the x descent may be present because of this latter phenomenon ( Fig. 2.5 ). A c wave is sometimes observed after the a wave and is due to the sudden motion of the tricuspid annulus toward the right atrium at the onset of ventricular systole. The c wave follows the a wave at the same time as the PR interval on the ECG; thus first-degree AV block results in a more obvious c wave ( Fig. 2.6 ). When a c wave is present, the pressure decay following it is called an x descent.



The next pressure event is the v wave. There is often misunderstanding regarding the mechanism of the v wave. Although this event occurs at the same time as ventricular systole (and when the tricuspid valve is closed), the pressure rise responsible for the v wave is not due to ventricular systole but to passive venous filling of the atrium during atrial diastole. Conditions that increase filling of the right atrium create greater prominence of the v wave. The peak of the right atrial v wave occurs at the end of ventricular systole, when the atria are maximally filled, and corresponds with the end of the T wave on the surface ECG. The pressure decay that occurs after the v wave is the y descent and is due to the rapid emptying of the right atrium when the tricuspid valve opens. Atrial contraction follows this event and the onset of another cardiac cycle. In normal right atrial waveforms, the a wave typically exceeds the v wave. During inspiration the mean right atrial pressure decreases due to the influence of decreased intrathoracic pressure, and there is an augmentation of passive right ventricular filling; the y descents become more prominent ( Fig. 2.7 ).

Right Ventricular Waveform
The normal right ventricular systolic pressure is 20 to 30 mm Hg, and the normal right ventricular end-diastolic pressure is 0 to 8 mm Hg. Right ventricular tracings exhibit the characteristic features of ventricular waveforms with rapid pressure rise during ventricular contraction and rapid pressure decay during relaxation, with a diastolic phase characterized by an initially low pressure that gradually increases ( Fig. 2.8 ). The right atrial pressure should be within a few millimeters of mercury of right ventricular end-diastolic pressure unless there is tricuspid stenosis. With atrial contraction, an a wave may appear on the ventricular waveform at end diastole ( Fig. 2.9 ), which is not a normal finding because the normal, compliant right ventricle typically absorbs the atrial component without a significant pressure rise. Therefore the presence of a wave on a right ventricular waveform usually indicates decreased compliance as may be seen in patients with pulmonary hypertension, right ventricular hypertrophy, or volume overload.


Pulmonary Artery Waveform
The normal pulmonary artery systolic pressure is 20 to 30 mm Hg, and the normal diastolic pressure is 4 to 12 mm Hg ( Fig. 2.10 ). A systolic pressure difference should not exist between the right ventricle and the pulmonary artery, unless there is pulmonary valvular or pulmonary artery stenosis. The pulmonary artery pressure tracing is similar to other arterial waveforms, with a rapid rise in pressure, systolic peak, a pressure decay following peak ejection, and a well-defined dicrotic notch from pulmonic valve closure during the pressure decay culminating in a diastolic trough. Peak systolic pressure occurs at the same time as the T wave on the surface ECG.

The pulmonary artery waveform, like other right heart chamber pressure waveforms, is subject to respiratory changes ( Fig. 2.11 ). Inspiration decreases intrathoracic pressure, and expiration increases intrathoracic pressure. The pressure changes associated with respiration transmitted to the cardiac chambers are often small and of little consequence. However, patients on mechanical ventilators with severe pulmonary disease, morbid obesity, or respiratory distress may generate substantial changes in intrathoracic pressure, resulting in marked differences in pulmonary artery pressures during the respiratory phases ( Fig. 2.12 ). Most experts consider end expiration to be the proper point to assess pulmonary artery (and other cardiac chamber) pressures because it is at this phase that intrathoracic pressure is closest to zero. If this effect is prominent and makes interpretation difficult, many operators will ask the patient to pause their breathing at end expiration during pressure sampling. The pulmonary artery end-diastolic pressure is sometimes used as an estimation of the left atrial pressure; however, it is highly inaccurate, especially if the pulmonary vascular resistance is abnormal.


Novel Methods of Measuring Pulmonary Artery Pressure in the Patient With Heart Failure
Invasive hemodynamic assessments are immensely helpful in understanding cardiac disease processes and targeting appropriate therapy. However, these measurements are limited to a single “snapshot” in the cardiac catheterization laboratory, or occasionally, several days of monitoring in an intensive care unit. These may not accurately reflect the patient’s hemodynamic status at home under normal activities. Technology to allow long-term hemodynamic monitoring has been in development for years, including left atrial, right ventricular, and pulmonary artery pressure sensors. Currently, there is approval and clinical use of the CardioMEMS (Abbott, Chicago, Illinois) pulmonary artery pressure sensor. This device is implanted into a branch of a pulmonary artery ( Fig. 2.13 ) and calibrated by simultaneous invasive measurements. Subsequent measurements are obtained from the pulmonary artery pressure sensor by an external receiver, generating a pulmonary artery waveform ( Fig. 2.14 ), which is transmitted to the care team for review and intervention. The interpretation software allows the review of pulmonary artery pressure trends over time ( Fig. 2.15 ). Based on the results of several clinical trials, the device is approved in the United States and is indicated to reduce heart failure hospitalizations in patients with New York Heart Association Class II–III symptoms with prior hospitalization for heart failure or elevation of brain natriuretic peptide. Heart rate and arrhythmia information is easily obtained from the waveforms by manual inspection, and algorithms to monitor cardiac output are in development.



Pulmonary Capillary Wedge Pressure Waveform
The normal mean pulmonary capillary wedge pressure (PCWP) is 2 to 14 mm Hg ( Fig. 2.16 ). A true PCWP can be measured only in the absence of anterograde flow in the pulmonary artery and with an end-hole catheter, such that pressure is transmitted through an uninterrupted fluid column from the left atrium, through the pulmonary veins and pulmonary capillary bed, to the catheter tip wedged in the pulmonary artery. Under these circumstances, the PCWP is a reflection of the left atrial pressure with a and v waves and x and y descents.

The PCWP tracing exhibits several important differences from a directly measured atrial pressure waveform. The c wave is absent because of the damped nature of the pressure wave. The v wave typically exceeds the a wave on the PCWP tracing. Because the pressure wave is transmitted through the pulmonary capillary bed, a significant time delay occurs between an electrocardiographic event and the onset of the corresponding pressure wave. The delay may vary substantially, depending on the distance the pressure wave travels. Shorter delays are observed when the PCWP is obtained with the catheter tip in a more distal location. Typically, the peak of the a wave follows the P wave on the ECG by about 240 ms, rather than 80 ms as seen in the right atrial tracing. Similarly, the peak of the v wave occurs after the T wave has already been inscribed on the ECG. The relationship between true left atrial pressure and PCWP is shown in Fig. 2.17 . Note the time delay between the same physiological events and the “damped” nature of the PCWP relative to the left atrial waveform, with a pressure slightly lower than the left atrium it is meant to reflect. In general, the mean PCWP is within a few millimeters of mercury of the mean left atrial pressure, especially if the wedge and pulmonary artery systolic pressures are low. High pulmonary artery pressure creates difficulty in obtaining a true “wedge,” falsely elevating the PCWP relative to the left atrial pressure.

Obtaining an accurate and high-quality PCWP tracing is not always easy or possible. An uninterrupted fluid column between the catheter tip and the left atrium is important. However, the lung consists of three distinct physiological pressure zones, with a different relationship between the alveolar, pulmonary artery, and pulmonary venous pressures (the lung zones of West) ( Fig. 2.18 ). Zone 1 is typically present in the apex of the lungs, where the alveolar pressure is greater than the mean pulmonary artery and pulmonary venous pressures. Zone 2 is located in the central portion of the lung, and pulmonary artery pressure exceeds alveolar pressure, which, in turn, is greater than the pulmonary venous pressure. These zones are not acceptable for estimation of the PCWP because capillary collapse is present based on these pressure relations, and a direct column of blood does not exist between the left atrium and the wedged catheter tip. Lung zone 3 is represented by the base of the lung, where alveolar pressure is lower than both pulmonary arterial and pulmonary venous pressure, allowing pressure transmission directly from the left atrium to the wedged catheter tip. Lung zone 3 is where PCWP accurately reflects the left atrial pressure. Fortunately, in most patients in the supine position on a cardiac catheterization table, most of the lung is in zone 3. In addition, because most blood flows to that area, the catheter tip of a balloon flotation catheter usually ends up in zone 3. Situations associated with catheter tip location in a nonzone 3 location include the use of positive end-expiratory pressure (PEEP), mechanical ventilation (alveolar pressure is increased and less of the lung is zone 3), and hypovolemia. Demonstrating that the catheter tip is below the level of the left atrium, however, ensures a zone 3 location and greater accuracy.
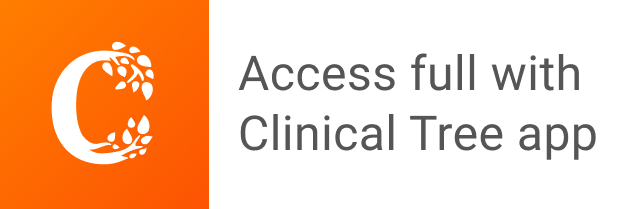