Fig. 1.1
Cardiac development: looping and septation. Schematic representation (a, b, d and f ) and electron microscopy images (c, e, g) of different stages of heart development, from heart fields (a) to a mature four-chambered septated heart (f ). In early developmental stages, bilateral cardiogenic heart fields (first and second heart fields) are present in the primitive plate (a). The structures derived from the second heart field are depicted in yellow whereas the structures derived from the first heart field are depicted in brown (a, b, d and f ). The primitive heart tube is formed after the fusion of the bilateral plates of mesoderm (b) from the first heart field (brown). The tube is lined with cardiac jelly (grey). A scanning electron microscopy (SEM) image of a comparable stage in chick heart development is depicted in (c). After looping of the heart has started, the different compartments of the heart can be recognized, the right portion of the ventricle (V) and the outflow tract (OFT) have started to form from the second heart field (yellow, d), while the left portion of the ventricle is derived from the first heart field (brown). A SEM image of a comparable stage in chick heart development is depicted in (e). Eventually, looping and septation as well as formation of the cardiac valves will be accomplished and the heart will have its mature adult form (f ). A SEM of a comparable stage in chick heart development is depicted in (e). A atrium, Ao aorta, AP arterial pole, LA left atrium, LV left ventricle, PT pulmonary trunk, RA right atrium, RV right ventricle, VP venous pole
Although this primitive heart tube was initially considered to be a miniature of the adult heart, more detailed data on early development of the heart were subsequently derived from animal studies including mouse and avian. These data correlate remarkably well with the descriptive work on human embryos from the early and mid-1990s [12–15]. Many descriptions and clarifications used here are based on animal experiments.
In the beginning of 2000, several studies brought prominently forward that the early embryonic heart tube was not the sole source of all components of the heart. This had already been established by Maria Victoria de la Cruz based on Indian ink injections in the embryonic avian heart tube [16]. The two best known papers to promote this concept are from the group of Roger Markwald [17] who describes the anterior heart field that adds myocardium to the outflow tract (OFT), while Margaret Kirby’s group describes almost the same population of cells and refers to this as the secondary heart field [18]. Thereafter, based on tracing studies using the LIM domain homeobox gene Isl1 as a marker of the late addition of myocardium to the heart tube, it turned out that the addition was taking place not only at the OFT but also at the inflow tract [19]. The term allotted to this wider spread mesodermal population, residing between the gut and the heart tube, was second heart field (SHF) as opposed to first heart field (FHF) being the source of the cells of the primary heart tube (Fig. 1.2). The use of the terms second and secondary heart field has led to some confusion. Our group designated the contribution of SHF to include both the posterior heart field providing the inflow tract, and the anterior heart field providing the OFT (Fig. 1.2). Remarkably, already in the primitive plate the areas of FHF and SHF can be designated [20] (Fig. 1.1). It should be noted, however, that although a distinction in nomenclature (i.e. FHF versus SHF) is generally made, both heart fields should probably be regarded as a spatiotemporal continuum, from which cells are progressively derived during cardiovascular development, with an earliest contribution to the primary heart tube.


Fig. 1.2
Schematic overview of cellular contributions to the developing heart. The left part of the figure depicts a schematic view of the primitive plate with mesenchyme of the first heart field (brown), second heart field (SHF, yellow) and (putative) neural crest cells (blue). The cellular contributions to the different compartments of the developing heart are schematically attached to the primitive plate. The first heart field (brown) gives rise to the primary heart tube (PHT) that contributes to the left ventricle (LV), atrioventricular canal (AVC) and part of the atria. During further development, cells are recruited to the heart from the SHF (yellow). The SHF can at the arterial pole of the heart be divided in the so-called secondary heart field, giving rise to the distal outflow tract (OFT; DOT) and in the anterior heart field (AHF) contributing to the proximal OFT (POT), the arterial pro-epicardial organ (aPEO) and the right ventricle (RV). At the venous pole of the heart, contributions are derived from the so-called posterior heart field (PHF), that supplies among other elements of the atria including the interatrial septum, cardiac conduction system (CCS), the myocardium surrounding the putative pulmonary and caval veins (PV & CV), as well as to the venous pro-epicardial organ (vPEO). An extracardiac contribution from the cardiac neural crest cells is depicted in blue. The right part of the figure shows cartoons of lateral views of an embryo during these processes in early (upper panel) and more progressed (lower panel) development. The PHT is lined on the inside by cardiac jelly (light blue). The mesoderm of the second heart field is depicted by the yellow area behind the primary heart tube, from which cells will be recruited to the heart tube at both the arterial and venous poles. As development proceeds (lower panel) segments of the heart will develop by progressive contribution of cells from the first and second heart field (yellow areas at the arterial en venous pole of the heart tube). BV brain ventricles, C coelomic cavity, DAo dorsal aorta, DMP dorsal mesenchymal protrusion, SAN sinoatrial node, G gut, ggL ganglia, IFT inflow tract, PAA pharyngeal arch arteries, SV sinus venosus. The right panel is published in [68] and reproduced with permission
The FHF is the source of the primitive LV, the atrioventricular canal myocardium and a small part of the atria, together constituting the primary heart tube. A very time laborious study of LaacZ cell tracing [21] revealed that almost the complete RV including the RV side of the ventricular septum is SHF derived [22] (indicated in yellow in Figs. 1.1 and 1.2). The RV OFT compartment shows a molecular distinction [23]. Together with observations on asymmetric distribution of Nkx2.5 positive SHF derived cells in the mesoderm of the OFT, we provide in this chapter novel data on the relative repositioning of the ascending aorta and pulmonary trunk.
Posterior heart field derived are the sinus venosus myocardium, encompassing the entrance of the superior and inferior caval vein and the coronary sinus. Recently, our concept was proven that the pulmonary veins also belong to this sinus venosus derived incorporation [24, 25].
Positioning of the OFT: The Pulmonary Push Concept
In the primary embryonic heart tube, the atrioventricular canal with the endocardial cushions completely connects to the primitive left sided ventricle. The OFT, initially situated entirely above the primitive RV, connects to the unseptated aortic sac that still needs to be divided into a pulmonary and aortic orifice leading to the great arteries. As mentioned above, during development the heart tube shows a dextral looping leading to a marked almost circular groove which is referred to as the inner curvature. The inner curvature is reflected internally by the primary fold or ring positioned between the primitive LV and the RV, which is still expanding due to the material addition of the SHF (Fig. 1.1). Both the atrioventricular canal myocardium and the nontrabeculated component of the OFT are lined by endocardial cushions. During remodelling and septation of the OFT, the aorta becomes connected to the LV. The long held idea was that rotation of the endocardial cushions was instrumental together with additional shortening of the subaortic OFT myocardium [26]. The mechanisms underlying rotation as well as the inferred apoptosis (programmed cell death) in the OFT have always remained hypothetical. We discovered an asymmetric and late contribution of Nkx2.5 expressing primitive mesenchyme (see Ref. [27]) on top of addition of smooth muscle cells preferentially to the pulmonary side of the OFT [28]. Analyzing this in 3D reconstructions we devised a new hypothesis in which rotational movement must be substituted for an anterior push of the subpulmonary myocardium resulting in lengthening of the RV OFT (Fig. 1.3 and Supplemental Video 1.1). This mechanism eliminates the requirement of marked subaortic apoptosis to understand the relative low position of the aortic orifice wedged between the tricuspid and the mitral orifice.


Fig. 1.3
Outflow tract positioning: the pulmonary push concept. 3D reconstructions of an embryonic day (E) 12.5 mouse embryonic heart. An anterior (a), right lateral (b) and posterior (c) view are depicted to elucidate the asymmetric contribution of Nkx2.5 positive mesenchyme (yellow) mainly to the left (putative pulmonary) side of the heart. Colour coding in the 3D reconstructions: red: aorta and left ventricle (LV) lumen; blue: pulmonary trunk (Pu), ductus arteriousus (DA) and right ventricle (RV) lumen; grey: transparent myocardium. OFT outflow tract. Modified after [28]
Tricuspid Orifice Formation
The dextral looping process and the relocation of the pulmonary orifice includes a marked relocation of the atrioventricular (AV) canal allowing the future tricuspid orifice to channel into the RV inflow tract compartment. Several concepts for this remodelling process have been proposed. Initially, the right side of the AV canal with the putative tricuspid orifice is positioned to the left of the primary fold (Fig. 1.1). The originally very small RV inflow tract enlarges and becomes positioned to the right of this fold. The exact mechanism in which this is achieved still remains elusive but concepts will be described in the section on ventricular septation.
Role of the Epicardium in Development of the Compact Myocardial Layer and Coronary Vascular Formation
The primary heart tube consists of an endocardial inner surface, a thick basement membrane, referred to as cardiac jelly, and an outer myocardial layer. During looping and SHF addition, the cardiac jelly persists in two endocardial cushion areas situated in the AV canal and in the OFT. Both cushion areas play a role in cardiac valve formation as well as in septation.
The remaining cardiac tube forming the future LV and RV is a two layered thin structure that borders on the outside to the coelomic cavity. Subsequently, two essential processes take place. First, the future outer compact myocardial layer and an inner trabecular layer become distinct, and second, progressive myocardial compaction needs to occur. The mechanism underlying trabecular formation, linked to AV valve development, has recently been described [29]. The system of trabeculations has an important mechanical function in the heart tube [30] maintaining ventricular wall strength. The trabecular sinuses also serve as a reservoir for blood. Before the complete development of the RV there is little difference in the trabecular architecture of the RV and LV [31] (Fig. 1.4). The compact layer of the myocardium still has to increase in thickness and interaction with the epicardium is an essential component of this process.


Fig. 1.4
Development of the compact myocardial layer. (a–d) Myocardial thickening and compaction of the right ventricular (RV) wall throughout mouse heart development: from embryonic day (E) 11.5 up to E14.5. A compact myocardial layer can be observed starting only at E14.5 in the RV. (e–h) Myocardial thickening and compaction of the left ventricular (LV) wall during the same stages of mouse heart development (E11.5–E14.5). Development of a compact myocardial layer can be observed starting from E12.5 and increasing in subsequent stages. (i and j) Overview sections of the heart at stage E13.5 in wild type (WT, i) and TGFβ2 knockout (−/−, j) embryos. In TGFβ2 knockout embryos thin myocardium is observed in both the LV and RV. The interventricular septum (IVS) has a spongeous appearance. Bars: 100 μm
Most of the epicardium is derived from the so-called pro-epicardial organ (vPEO) positioned at the venous pole of the heart [32]. Recently we have shown that a second epicardial organ (aPEO) can be distinguished at the OFT (Fig. 1.2). Epicardial cells migrate from both sources over the heart (vPEO derived) and great arteries (aPEO derived). The anterior surface of the RV is the last to be covered in this process [33]. The epicardium becomes relatively dormant in adult life. However, the role of the epicardium in cardiac development has received the attention of many research groups because myocardial injury is associated with adult reactivation, which may play a role in repair after myocardial infarction, Several recent reviews cover this area [32, 34–36] containing the information on the underlying primary research.
The data that reflect specifically on the development and morphology of the RV will be presented here. The epicardium derived from the vPEO is referred to as cardiac epicardium (cEP) as it covers only the myocardium up to the ventriculo-arterial junction. After this covering a process of epithelial-mesenchymal-transition (EMT) leads to a population of epicardium derived cells (EPDCs) that move into the subepicardial space and subsequently migrate into the myocardium. Many genes and molecular pathways are involved in this process [37]. Having entered the myocardium, EPDCs will differentiate into the smooth muscle cells of the coronary arteries and into intracardiac fibroblasts. It cannot be excluded that these populations are already programmed to their fate while still being on the surface [38] which might, therefore, harbour a heterogeneous epicardial population. When epicardial outgrowth [39], the process of EMT or migration are disturbed [32], normal compaction of the ventricular myocardium will not take place leading in the most severe cases to a very thin compact myocardium or embryonic death. This has been described in many animal models based on mutated genes influencing the epicardium or the ensuing cross-talk between epicardium and myocardium (an example is shown in Fig. 1.4 for a TGFβ mutant mouse). This important population for myocardial differentiation shows a temporo-spatial difference in the contribution to the RV and LV wall (unpublished data). In summary (1) the EPDC migration into the RV precedes slightly the migration into the LV wall, (2) eventually the LV receives more EPDCs and (3) there is a difference in anterior to posterior deposition of EPDCs in the RV wall. The RV receives relatively more EPDCs than the LV, and in both ventricles the posterior wall holds more EPDCs than the anterior wall. Whether this observation is relevant for adult RV and LV function is not known but it is tempting to relate it to non-compaction cardiomyopathies and the difference between RV and LV types. In several forms of congenital heart disease, the posteriorly situated inflow compartment is mostly affected, as is described for pulmonary atresia with intact ventricular septum resulting in a bi- or unipartite ventricle.
The EPDCs that differentiate into smooth muscle cells start to cover the main arterial stems that have grown into the aorta at specific sites of the aortic wall [40, 41]. These initial observations in avian embryos are now confirmed in mouse studies [42]. The reason that normally the coronary vessels do not penetrate the pulmonary wall is postulated to relate to the specific myocardial origin of the subpulmonary OFT.
Disturbed epicardial contribution can lead to diminished coronary vascularization of the myocardium as well as absent or pin-point coronary arterial orifices [41, 43]. The relevance for specific RV pathology might relate to the incompletely understood preference of ventriculo-coronary-arterial communications (VCACs) or fistulae in case of pulmonary atresia without ventricular septal defect (VSD) in the hypoplastic RV [7, 8]. A similar coronary arterial pathology is not seen in the hypoplastic left heart [44]. It is unclear whether myocardial architecture dictates distribution of the microvascular coronary capillaries or vice versa. It is obvious that the normal LV has a more regular patterning of capillaries compared to the RV [45].
Morphology Right Versus Left Ventricle (Fig. 1.5)
The RV morphology is clearly different from the LV based on a number of structural characteristics of the RV cavity. The entrance into the RV from the right atrium is via the tricuspid orifice which is encircled by a fibrous annulus composed of fine collagen. The tricuspid valve consists of three valve leaflets: the posterior, the anterior and the septal leaflet. These leaflets are attached by chordae tendineae to the papillary muscles including the septal and moderator band. In general the RV is divided into three parts: the tripartite division [46]. Following the flow, these consist of (1) a posteriorly located inlet segment. Subsequently, crossing the septal to moderator band continuity (Fig. 1.5) (2) the trabecular component reaching up to the apex. The latter apical compartment is lined by relatively coarse trabeculations that distinguish the RV from the finely trabeculated LV. The last compartment is (3) the relatively smooth walled OFT leading to the pulmonary orifice and pulmonary trunk. The pulmonary orifice harbours three semilunar valve leaflets. The anchorage of this orifice into the OFT myocardium differs from the way into which the aortic orifice is embedded into the LV [47–49].


Fig. 1.5
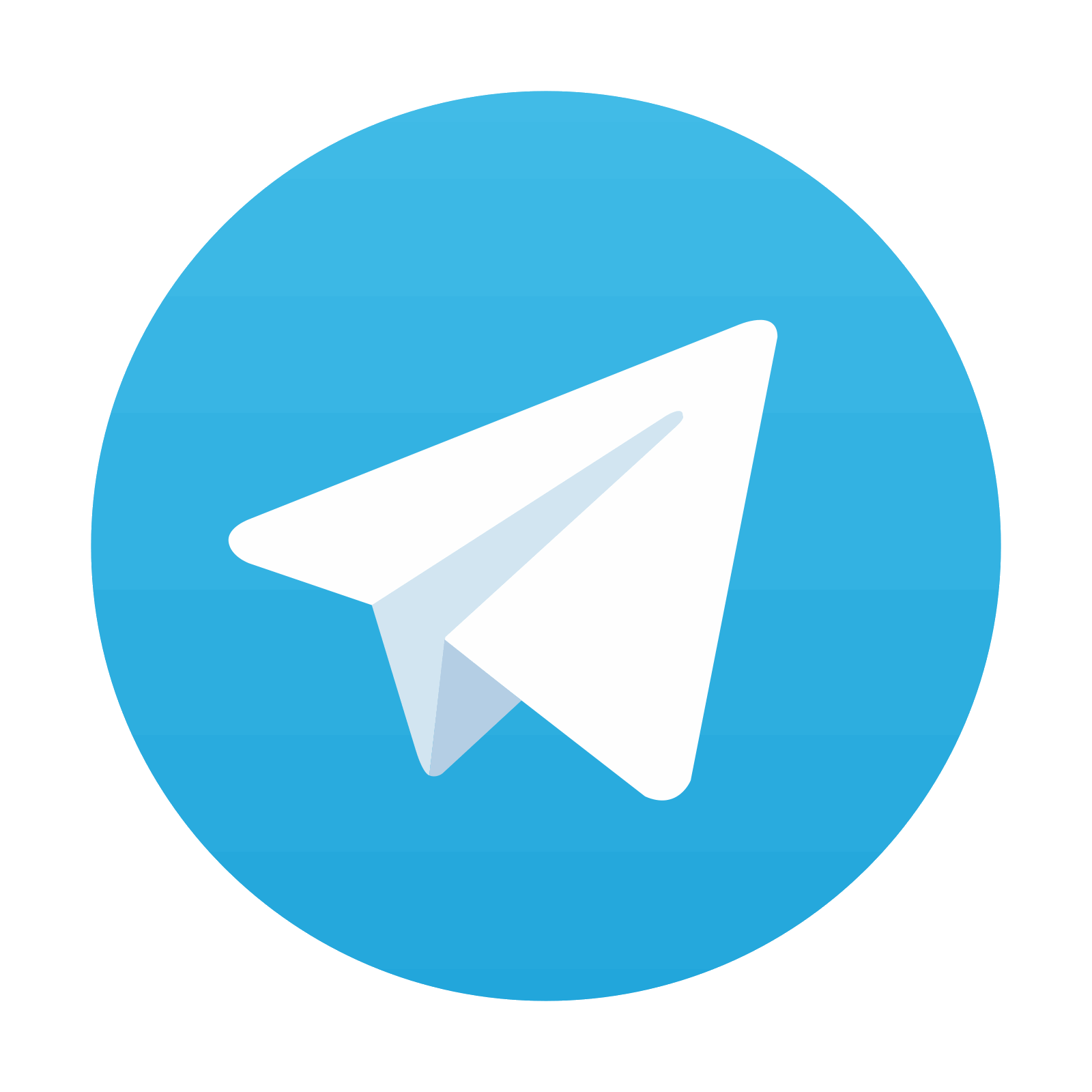
Ventricular morphology. (a–d) Pictures of a neonatal heart of 36 weeks gestation (a) Frontal and (b) superior view, showing the normal left anterior position of the pulmonary trunk (PT) as related to the aorta (Ao). (c) View into the right ventricle (RV). The tricuspid valve (TV) is separated from the orifice of the pulmonary trunk (PT) by muscle tissue of the supraventricular crest, consisting of the ventriculoinfundibular fold (VIF) and the septal band (SB). The septal band (SB) is continuous with the moderator band (MB) that has been cut in this view. In the normal heart, the outflow tract septum cannot be recognized as a separate entity. (d) View into the left ventricle (LV). The mitral valve (MV) is in fibrous continuity with the aortic valve (Ao). The wall of ventricular septum (IVS) is smooth. (e) Drawing of the anatomical structures of the RV, showing the different compartments. The inlet septum (IS) is separated from the trabeculated apical part by the septal band (SB). The part above this continuity towards the PT is the outflow part of the RV. (f) Adult heart, in which the RV has been fenestrated to demonstrate the moderator band (MB), that crosses the RV lumen from the IVS towards the lateral wall. (g) Close up of the MB. (h) Neonatal heart with two ventricular septal defects (VSD): the upper defect (indicated by one probe) is a perimembranous VSD, characterized by a fibrous continuity in the lower rim of the defect. The lower defect (indicated by two probes) is a central muscular VSD. A case of transposition of the great arteries with a malalignment VSD (probe) where the outlet septum (asterisk) is malaligned with the remainder of the IVS and thus can be recognized as structure separated from the ventriculoinfundibular fold (VIF) and the septal band. The pulmonary orifice (PT) overrides the septum and is partly above the RV
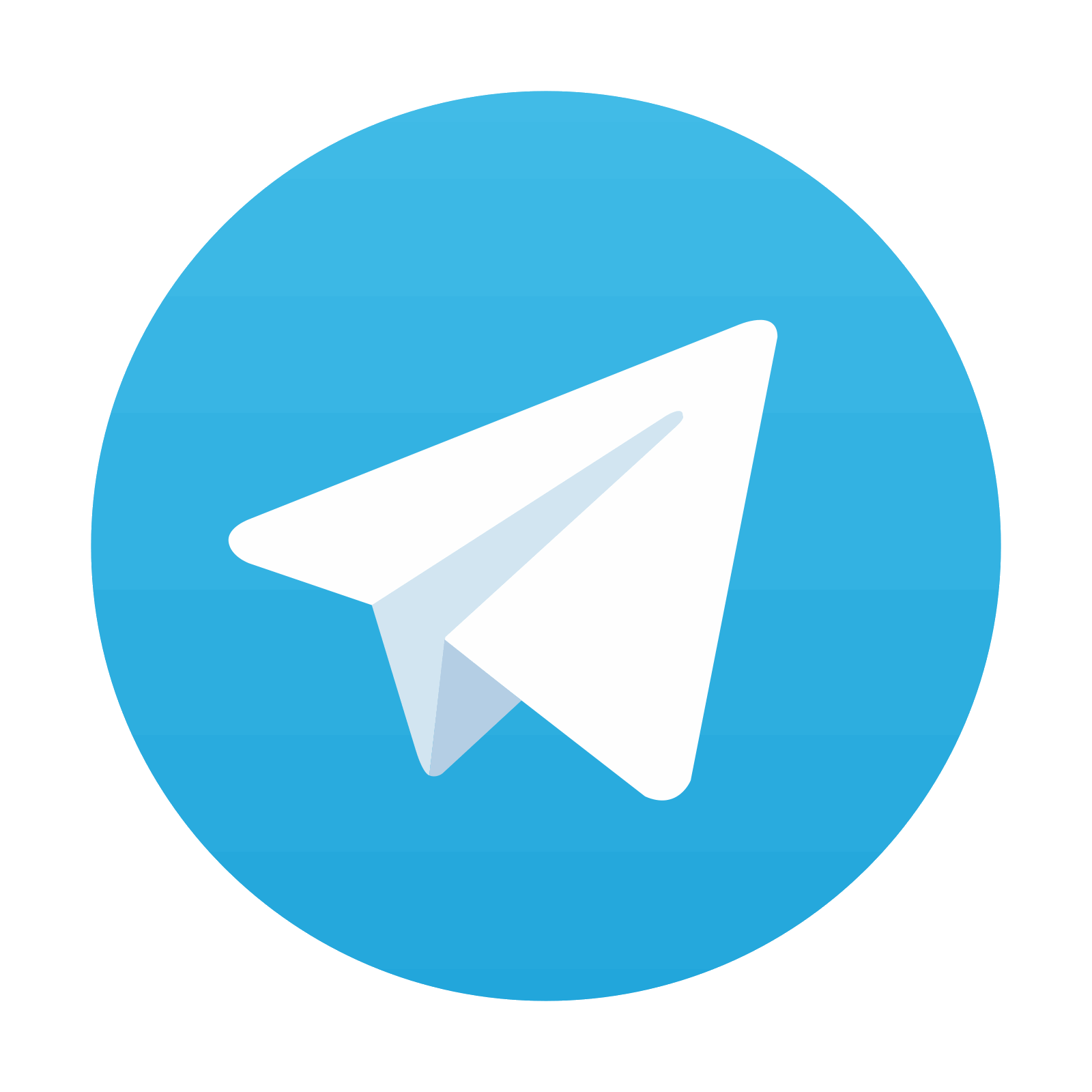
Stay updated, free articles. Join our Telegram channel
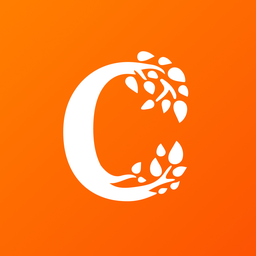
Full access? Get Clinical Tree
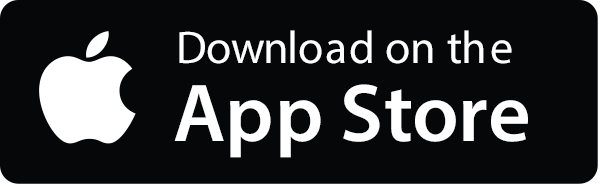
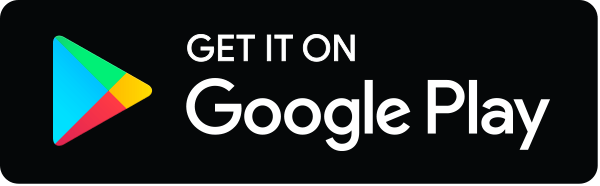
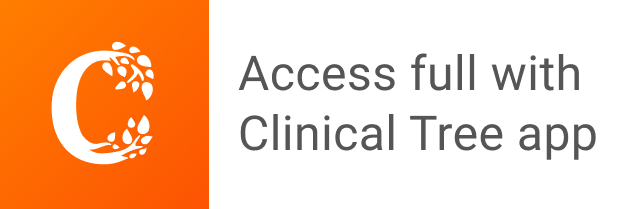