Mathematically describing fluid systems is very complex. Hemodynamics can be defined as the physical factors that influence blood flow that are based on fundamental laws of physics, namely Ohm’s law. voltage (ΔV) equals the product of current (I) and resistance (R).
In relating Ohm’s law to fluid flow, the voltage is the pressure difference between two points (ΔP), the resistance is the resistance to flow (R), and the current is the blood flow (F).
Resistance to blood flow within a vascular network is determined by the length and diameter of individual vessels, the physical characteristics of the blood (viscosity, laminar flow versus turbulent flow), the series and parallel arrangements of vascular network, and extravascular mechanical forces acting upon the vasculature. This is expressed in Poiseuille’s law:
Poiseuille’s Law relates the rate at which blood flows through a small blood vessel (Q) with the difference in blood pressure at the two ends (ΔP), the radius (r) and the length (L) of the artery, and the viscosity (η)of the blood.
Of the above factors, changes in vessel diameter are most important quantitatively for regulating blood flow. Changes in vessel diameter, either by constriction or dilatation, enable organs to adjust their own blood flow to meet the metabolic requirements of the tissue.
Osborne Reynolds quantified how viscosity, vessel radius, and pressure/volume relations influenced the stability of flow through a vessel.
Density and viscosity are relatively constant; therefore, the development of turbulence depends mainly on the velocity and size of the vessel. Density is defined as mass per unit volume, and viscosity is defined as a measure of the resistance of a fluid to being deformed by either shear stress or extensional stress. A Reynolds above 2000 causes turbulence and vessel wall vibration, producing a bruit. High velocities cause turbulence and hinder volumes flow, creating eddies.
The anterior tibial veins drain blood from the dorsum of the foot (dorsalis pedis veins) and anterior compartment of calf. The anterior tibial veins originate near the tibia at the ankle, lying anterior to the interosseous membrane as they ascend the lower leg and move toward the fibula. The anterior tibial vein joins the posterior tibial vein to become the popliteal vein. The posterior tibial vein originates from the plantar (superficial and deep) veins of the foot. They run from the medial malleolus along the medial calf with the posterior tibial artery. The posterior tibial veins drain blood from the posterior compartment of the lower leg. They receive the combined peroneal vein (made up of the tibial and peroneal) to become the popliteal vein. The peroneal veins course along with the peroneal artery near the fibula. The peroneal vein drains blood from the lateral aspect of the lower leg. These veins lie deep to the soleus and parallel to the path of the posterior tibial vein. These deep veins are generally paired.7
Lying deep within the muscular compartment of the calf course the sural veins and soleal sinuses. The soleal sinuses are large venous reservoirs that lie within the soleus muscle and empty into the posterior tibial or peroneal veins. The gastrocnemius veins are paired and accompany the artery, which lies medial and lateral to the gastrocnemius muscle. These terminate into the popliteal vein. The popliteal vein accompanies the popliteal artery (confluence of anterior tibial and tibial–peroneal trunk), and lies superficial to the artery. The femoral vein originates at the hiatus of the adductor magnus muscle in distal thigh and ascends through Hunter’s canal (adductor) and courses deep to the artery and terminates in the femoral triangle. The femoral vein previously was called the superficial femoral vein, but this nomenclature has been abandoned by most clinical practitioners and anatomists as confusing because it is a deep vein.7
The deep femoral (profunda femoris) vein joins the femoral vein to form the common femoral vein. The common femoral vein lies in Scarpa’s triangle medial to the artery, receives the great saphenous vein, and terminates at the level of the inguinal ligament to become the external iliac vein. The external iliac vein joins the internal iliac vein to form the common iliac vein. The right and left common iliac veins join to form the inferior vena cava. The left common iliac vein crosses beneath the right iliac artery, which in some individuals can cause compression of the left common iliac vein, creating an increased risk of left-sided deep venous thrombosis (DVT; May-Thurner syndrome).
The great saphenous vein originates on the dorsum of the foot and ascends anterior to the medial malleolus and along the anteromedial side of the calf and thigh, eventually joining the common femoral vein. The small saphenous vein originates on the dorsum of the foot and ascends posterior to the lateral malleolus and runs midline to the posterior calf, terminating as it joins the popliteal vein.
The posterior arch vein is a main tributary of the great saphenous vein posterior to medial malleolus and terminates in the great saphenous vein below the knee. Perforating veins connect the superficial and deep veins near the ankle, in the calf, and in the lower thigh.
The veins of the upper extremities include the deep veins from the paired radial and ulnar veins. The axillary vein is formed when the basilic vein joins the brachial veins (most often a pair) in the upper arm at the lower border of the teres major and terminates beneath the clavicle on the outer border of the first rib. The proximal axillary vein receives blood from the cephalic vein.8,9,10
The subclavian vein is a continuation of the axillary vein (outer border of first rib to inner end of clavicle) and joins the internal jugular vein to form the innominate vein. The right internal jugular vein receives blood from the brain, right vertebral, internal mammary, and inferior thyroid veins. The left internal jugular vein receives blood from the left vertebral, internal mammary, inferior thyroid, and left superior intercostal veins. The right superior intercostals veins drains into the azygous vein. The innominate veins then empties into the superior vena cava.9,11
The cephalic vein begins on the dorsum of the hand, courses along the bicep and along the deltopectoral grove, and penetrates the deep fascia to join the axillary vein. The basilic vein courses from the small fingers of the dorsum of the hand, courses medially along with the biceps, and joins with the brachial veins in forming the axillary vein.
Ultrasonic waves entering human tissue are absorbed, reflected, and scattered to produce images of anatomic structures. The transmission properties of the sound waves depend on the density and elasticity of the tissues. The density and speed of propagation of ultrasound waves determine a tissue’s acoustic impedance. The larger the differences in acoustic impedance between tissues, the more ultrasound waves are reflected. The reflection further depends on the angle of insonation. Strong reflective interfaces such as air or bone prevent imaging of weaker echoes from deeper tissue and cast shadows behind them. Whereas tissues that strongly reflect ultrasound are termed hyperechoic, poorly reflective tissues are termed hypoechoic, and isoechoic tissues contain the same relative echogeneity as those of immediately adjacent structures.
The transducer repeatedly emits pulses of sound at a fixed repetition frequency. A detector records echoes originating from interfaces and scatters in the sound beam. Echo signals are then amplified and processed into a format for display. If ultrasound is continuously transmitted along a particular path, the energy will also be continuously reflected back from any source in the path of the beam, making it difficult to predict the depth of the returning echoes. With pulse-echo techniques, it is possible to predict the distance of a reflecting surface from the transducer if the time between the transmission and reception of the pulse is measured and the velocity of the ultrasound along the path is known.
When the ultrasound pulse returns to the transducer, it causes the transducer to vibrate and will generate a voltage across the piezoelectric element. The amplitude of the returning pulse depends on several factors, including the proportion of the ultrasound reflected to the transducer and by which the signal has been reflected along its path. The amplitude of the pulse received back to the transducer can be displayed (A-mode) against time and then can be calibrated to time, thus showing the depth of the boundary in the tissue. The varying in amplitude of the signal can be displayed as a spot of varying brightness. This type of display is known as a B-mode scan.
Structures imaged by B-mode, or brightness mode, are displayed proportionally to the intensity of returning echoes. The ultrasonic beam scanning through a tissue plane produces a two-dimensional gray-scale image. In clinical practice, the beam is swept quickly through the field of view, and the image is continuously renewed, allowing visualization of the underlying tissue anatomy.
B-mode ultrasound takes reflected signals and converts them to a series of dots on a display. A transducer, whose resonant frequency is between roughly 2 and 10 MHz, is used to transmit a short pulse of sound into a patient. The sound is reflected from a tissue interface where there are differences in acoustic impedance. The reflected pulse is received by the ultrasound instrument, and the pulse amplitude is encoded as brightness and depth on a monitor. The time that is required for the pulse to travel from the transducer to the interface and back is directly proportional to its depth. The acoustic velocity in blood is 1540 m/s. As the sound pulse propagates through tissue, it is attenuated, darkening the parts of the image that correspond to regions further from the probe. To compensate for this attenuation, echoes originating from deeper tissues are amplified more than echoes originating near the probe. Because different tissues have different attenuation coefficients, the amplification can be varied as a function of depth. The exact manner in which the amplification depends on depth is usually displayed on the ultrasound instruments monitor as a depth–gain curve or time–gain curve. Usually, 100 to 200 separate ultrasound beam lines are used to construct each image.
Doppler ultrasound refers to the change in frequency when the motion of laminar or turbulent flow is detected within a vascular structure. In the medical application of the Doppler principle, the sound wave is bounced off a moving red blood cell (RBC). If the cell moves along the line of the ultrasound beam (parallel to flow), the Doppler shift is directly proportional to the velocity of the RBC. If the cell moves away from the transducer in the plane of the beam, the fall in frequency is directly proportional to the velocity and direction of RBC motion.
Doppler ultrasound is a technique for making noninvasive velocity measurements. The difference in frequency between emitted and returning ultrasonic echoes is the Doppler frequency shift.1 Because the blood is moving, the sound undergoes a frequency (Doppler) shift that is described by the Doppler equation1,2:
where c is the acoustic velocity in blood, 1540 m/s, Fo is the transmitted frequency, θ is the Doppler angle, and v is the velocity of the blood. The shift is measured only for the component of motion occurring along the ultrasound beam. Therefore, absolute velocity measurements require that a correction be made for the angle (θ) between the vessel and the beam as follows:
Color Doppler ultrasonography is a technique for visualizing the velocity of blood within an image plane. Color is superimposed on a conventional gray-scale image to enhance the image of the Doppler frequency shift. A color Doppler instrument measures the Doppler shifts in a few thousand sample volumes located in an image plane. For each sample volume, the average Doppler shift is encoded as a color and displayed on top of the B-mode image. The way the frequency shifts are encoded is defined by the color bar located to the side of the image. By convention, positive Doppler shifts, caused by blood moving toward the transducer, are encoded as red, and negative shifts are encoded as blue. Color Doppler images are updated several times per second, allowing the flowing blood to be easily visualized. Velocities are quantified by allocating a pixel to flow toward the transducer and flow away from the transducer. Each velocity frequency change is allocated a color. Color maps may be adjusted to obtain different color assignment for the velocity levels; red is usually flow toward the transducer, and blue is flow away from the transducer.3
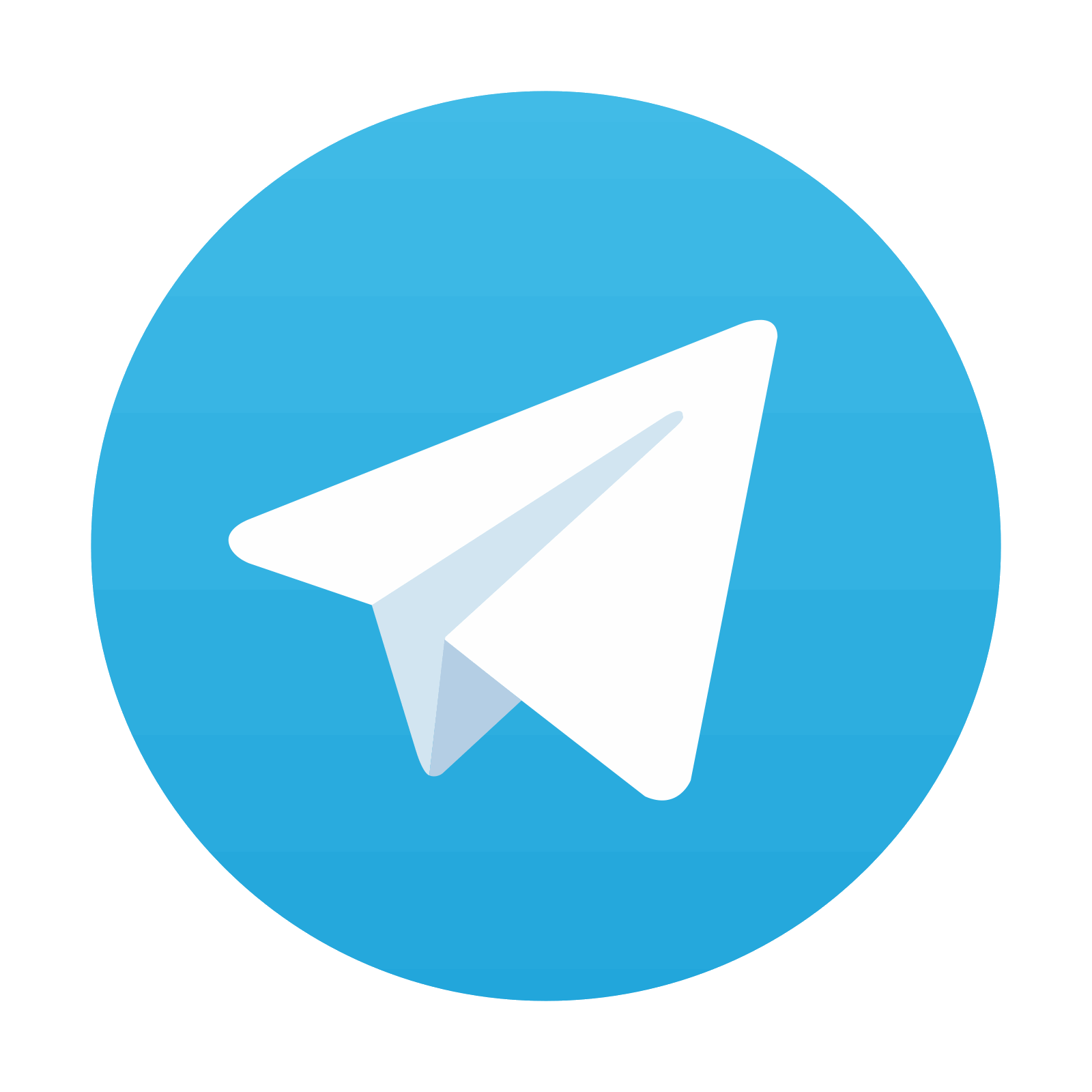
Stay updated, free articles. Join our Telegram channel
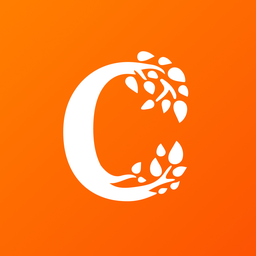
Full access? Get Clinical Tree
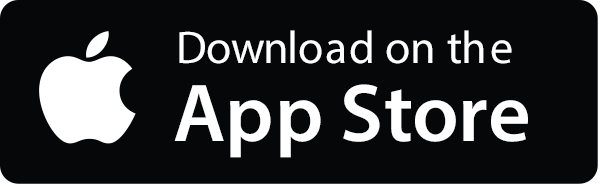
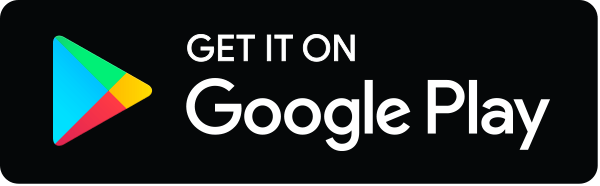