Chapter 11 Non-invasive ventilation
INTRODUCTION
The application of non-invasive ventilatory support to improve ventilation is not a new idea. The tank ventilator or ‘iron lung’, which provides negative pressure to the chest wall, was first developed in the 19th century (Woollam 1976). Further developments and modifications occurred, but it was not until the poliomyelitis outbreaks of the 1940s and 1950s that such devices became widely used. Continuous positive airway pressure (CPAP) through a facemask for patients with pulmonary oedema and other forms of acute respiratory failure was extensively described in the 1930s (Barach et al 1938). However, with the development of positive pressure ventilators and the introduction of the endotracheal tube in the 1960s, use of non-invasive forms of ventilatory support for acute respiratory failure declined. Negative pressure devices continued to be used in patients with severe respiratory muscle impairment following poliomyelitis and in other patient groups presenting with chronic respiratory failure where long-term ventilatory support in the home was required (Garay et al 1981, Weirs et al 1977).
Since the mid-1980s, interest in non-invasive ventilatory support has again flourished, specifically the use of positive airway pressure devices and facemask interfaces. Although this interest had its genesis in the area of sleep-disordered breathing and chronic respiratory failure, clinicians have rapidly recognized the value of this therapy in acute medical and surgical conditions where respiratory failure develops, in weaning from conventional ventilatory support and as an adjunct to established respiratory care programmes. In this chapter we will outline the mechanisms by which abnormal sleep-breathing contributes to the development of awake respiratory failure and the place nocturnal ventilatory support plays in reversing this. We will also look at the potential application of this technique in a broadening range of clinical conditions.
BREATHING, SLEEP AND RESPIRATORY FAILURE
It has been recognized for many years that significant changes in breathing and ventilation can occur during sleep (Gastaut et al 1966). The contribution abnormal breathing, during sleep, can play in the development of awake hypercapnia is now more fully appreciated. Our understanding of what happens to breathing during sleep has been greatly enhanced by three major developments in technology:


Changes in breathing during sleep
The awake state itself is associated with an additional stimulus to breathe, over and above that determined by the metabolic control system. This is known as the wakefulness drive to breathe and is lost with the onset of sleep. General postural muscle tone is also reduced at sleep onset, resulting in increases in upper airway resistance and reductions in ventilatory drive. At the same time, ventilatory responses to both hypoxia and hypercapnia are reduced so that there is an attenuated response to changes in gas exchange compared with wakefulness. As a result, a small fall in ventilation occurs with sleep in the range of 10–15% (Douglas et al 1982).
Although reduced, ventilation during non-rapid eye movement (NREM) sleep is steady, particularly during periods of slow-wave sleep. However, even in normal subjects there is substantial variation in breathing during rapid eye movement (REM) sleep, most pronounced during periods of phasic eye movements. During these episodes, alveolar ventilation may fall by as much as 40% (Douglas et al 1982). REM sleep is also associated with alterations in respiratory control, caused by descending inhibition of alpha and gamma motor neurons. This produces hypotonia of postural muscles, including the intercostal and accessory respiratory muscles and a reduction in the rib cage contribution to ventilation. As a result, ventilation during REM sleep becomes heavily reliant on diaphragmatic activity.
The role of sleep in the development of awake hypercapnic respiratory failure
It is now well recognized that disturbed breathing first becomes apparent in REM sleep (Bye et al 1990). However, as REM sleep takes up only a relatively small proportion of total sleep time, patients with REM hypoventilation, even if severe, may remain clinically stable for months or even years before significant daytime hypercapnia becomes apparent. Initially, ventilation and sleep between periods of REM hypoventilation are usually normal, often through the recruitment of accessory respiratory muscles. In addition, the arousal mechanism operates to defend ventilation by limiting the amount of time spent in REM sleep and therefore the degree of abnormal gas exchange that occurs. Characteristically, awake blood gases remain normal during this initial stage.
Progression of abnormal breathing into NREM sleep heralds the second stage in the evolution of sleep-induced respiratory failure (Piper & Sullivan 1994a). Mechanisms responsible for this progression include not only a deterioration of the underlying disease itself but also the appearance of other factors that may load breathing such as ageing, weight gain, upper airway dysfunction or the development of an intercurrent illness such as a chest infection. Sleep fragmentation from abnormal breathing events has the capacity to further alter respiratory control and depress arousal. These factors allow more severe sleep-disordered breathing to occur, with less arousal between events. This begins a vicious cycle whereby resetting the sensitivity of the ventilatory control system occurs so that higher levels of carbon dioxide and lower levels of oxygen are tolerated without stimulating a change in respiration, not only asleep but during wakefulness as well. During this stage, daytime CO2 retention becomes apparent (Fig. 11.1).
The final stage in the development of sleep-induced hypercapnia is characterized by unstable respiratory failure both awake and asleep. During this stage, changes in blood gases during sleep are extreme and sleep architecture may be profoundly disturbed. By this stage, the clinical condition of the patient may deteriorate considerably, which can be mistaken for a progression of the underlying disease process. It is crucial that clinicians recognize patients with nocturnal hypoventilation early and treat appropriately, in order to prevent the complications associated with an acute respiratory crisis (Box 11.1).
Box 11.1 Common conditions where nocturnal hypercapnic respiratory failure is likely to occur
Neuromuscular | Myopathies Neuropathies |
Chest wall | |
Impaired ventilatory | Brainstem injury |
control | Primary alveolar hypoventilation |
Airway obstruction | Severe obstructive sleep apnoea |
Lung disease |
NON-INVASIVE VENTILATION
Rationale and methods
In patients presenting with acute respiratory failure there is a deterioration in gas exchange accompanied by changes in pulmonary mechanics with increased respiratory loads. As a consequence a shallow, rapid breathing pattern develops with shortening of inspiratory time and reductions in tidal volume. Non-invasive ventilatory support in this setting aims to augment the patient’s tidal volume while reducing the amount of effort or work performed. In addition, maintaining a low level of positive pressure during expiration can subsequently reduce inspiratory effort by counterbalancing intrinsic PEEP (the end-expiratory recoil pressure of the respiratory system due to incomplete expiration). The improved breathing pattern with NIV should improve alveolar ventilation while preventing respiratory muscle fatigue. Ventilators that provide a range of settings and features can be desirable in the acute situation in order to ensure the machine has the capability of addressing the individual’s breathing needs.
The mechanism by which NIV improves awake spontaneous breathing in patients with chronic respiratory failure is a little less clear. Three possible hypotheses have been put forward (Hill 1993). The first relates to chronic muscle fatigue and proposes that the use of NIV permits intermittent rest of fatigued muscles, restoring function. The second hypothesis proposes that NIV increases lung volumes and compliance. Finally, the respiratory centre sensitivity to CO2 may be blunted during the development of chronic respiratory failure. Non-invasive ventilation, by preventing hypoventilation during sleep, may work by restoring ventilatory sensitivity to carbon dioxide and hence improve awake breathing. In a study designed to determine the relative importance of each of these mechanisms, Nickol et al (2005) found there was no change in measures of inspiratory muscle strength, lung function or respiratory system compliance with the commencement of NIV in patients with restrictive thoracic disease despite significant improvements in awake blood gases. However, they did find an increase in ventilatory sensitivity to CO2 supporting this as a principle mechanism for the improvements in breathing seen in patients with chronic respiratory failure who had been started on NIV.
Ventilator systems
Volume-preset machines such as the PLV®-102 (Respironics, Inc, Murrysville, PA, USA), the PV501 (BreasTM, Molnycke, Sweden) or the BromptonPAC (PneuPAC Ltd, Luton, Beds, UK) operate as time-cycled flow generators and deliver a fixed tidal volume irrespective of the airway pressure generated, provided that leaks from the system are minimized. Pressure-preset systems include bilevel positive pressure devices, the most widely recognized being the BiPAP® machines (Respironics, Inc, Murrysville, PA, USA). Other pressure-preset devices include the Vivo30® (BreasTM, Molnycke, Sweden), VS IntegraTM (ResMed, Bella Vista, NSW, Australia), and the VPAPTM III device (ResMed, Bella Vista, NSW, Australia) (Fig 11.2A). With these devices, tidal volume will vary according to the preset inspiratory pressure, the inspiratory–expiratory pressure difference and the chest wall and lung compliance of the patient. Bilevel devices are reported to compensate better for mild to moderate leaks than volume-preset devices (Mehta et al 2001). However, leaks are common during mask ventilation, particularly during sleep, and this may adversely affect the quality of ventilation and sleep architecture even with bilevel positive pressure devices (Piper & Willson 1996, Teschler et al 1999).
In studies comparing the efficacy of these two systems, little difference has been found either in acute (Girault et al 1997) or chronic respiratory failure (Tuggey & Elliott 2005), although many patients find pressure preset devices easier to tolerate. Poor tolerance to volume-preset devices may be related to an increase in airway resistance causing an elevation of inspiratory pressure in the mask, which may be uncomfortable or may cause leaks, thus limiting the effectiveness of ventilation. On the other hand, in patients with low chest wall compliance, higher airway pressures may be needed to maintain optimal ventilation. In these patients, volume-preset ventilators can prove more reliable and effective in delivering a stable tidal volume despite changing chest wall mechanics or airway resistance. A change to a volume-preset device should always be considered if hypoventilation persists on bilevel ventilatory support (Schonhofer et al 1997).
Criteria for choosing mode of ventilatory support
Sleep study data are useful in patients requiring long-term ventilation in identifying any degree of upper airway dysfunction that may be present as well as determining the patient’s respiratory drive during sleep. Understanding the features and limitations of the various machines available and the modes of ventilatory support in which they can operate will assist in selecting the appropriate system to meet the patient’s needs. A recent development is the availability of portable ventilators that are able to deliver both pressure and volume ventilation, allowing flexibility with regard to changing the mode of ventilatory sup-port with changes in the patient’s condition (e.g. PLV® ContinuumTM (Fig. 11.2B), Respironics, Inc, Murrysville, PA, USA; PV403, BreasTM, Molnycke, Sweden; VS UltraTM, ResMed, Bella Vista, NSW, Australia).
Settings
The mode of support needs to be set so that the breaths delivered will be either machine-triggered or patient-triggered. With bilevel devices, a spontaneous mode of support is available, where the machine cycles into inspiration in response to the patient’s spontaneous inspiratory effort. The volume-preset and a number of the bilevel devices can also be set to deliver a preset respiratory rate should the patient fail to trigger the device. Titration of the inspiratory positive airway pressure (IPAP) for a patient on a bilevel device or the tidal volume for a patient using a volume-preset device is made on the basis of patient tolerance and the effect such a setting has on ventilation and gas exchange. However, when setting pressures or volumes it should be borne in mind that excessively high inspiratory pressures will promote leakage of air from the mouth, reducing the effectiveness of ventilatory support. Excessive hyperventilation can also occur, which may induce upper airway obstruction and the appearance of central apnoea. The use of expiratory positive airway pressure (EPAP) may be advantageous in a number of clinical conditions, including controlling upper airway closure, recruiting collapsed alveoli or to overcome intrinsic end-expiratory pressure. Where inspiratory time or flow can be set, this will be based on the patient’s own respiratory pattern, taking into account the effect short inspiratory times can have on gas exchange.
Timing of intervention
There is extensive evidence to support the use of NIV as first-line therapy in patients with acute exacerbations of COPD (Lightowler et al 2003). However, the technique is not universally successful, with a proportion of patients continuing to deteriorate despite appropriate support. The likelihood of failure increases as acidosis worsens (Ambrosino et al 1995, Plant et al 2001). Instituting NIV in mild exacerbations has not been shown to be beneficial and may be poorly tolerated (Keenan et al 2003). Therefore, identifying and intervening during this window of therapeutic opportunity is important to achieve the best clinical outcomes with NIV. Generally speaking, patients with a pH in the range of 7.25–7.35 are the best candidates for the procedure.
One study looked at the ‘preventative’ use of NIV in patients with Duchenne muscular dystrophy (DMD), free of daytime respiratory failure (Raphael et al 1994). No benefit was found from early intervention with this technique, with the treated group showing a similar rate of deterioration in blood gases and pulmonary function as the control group. However, nocturnal monitoring was not performed either at baseline or during therapy in these patients. A more recent study looking at patients with neuromuscular disease, including patients with DMD, found that those with nocturnal hypoventilation despite daytime normocapnia were likely to deteriorate and develop daytime respiratory failure and/or progressive symptoms within 2 years if NIV was not introduced (Ward et al 2005). This suggests that the timing of NIV intervention is important, with identification of those patients at risk of developing nocturnal hypoventilation, monitoring of nocturnal gas exchange in these individuals and intervention with NIV before the development of awake respiratory failure ensues. In this way, the chances of the patient presenting with an acute respiratory crisis may be minimized.
INDICATIONS FOR NON-INVASIVE VENTILATION IN CHRONIC RESPIRATORY FAILURE
Assessment of chronic hypoventilation
Although a number of investigators have tried to use daytime pulmonary function tests as a predictor of the degree of abnormal breathing occurring during sleep, no strong correlation has been found (Bye et al 1990). However, it has been shown that a low vital capacity, a significant fall in vital capacity from erect to supine or a maximum inspiratory pressure of less than 30 cmH2O are all indicators that sleep-disordered breathing and hypoventilation may be present (Bye et al 1990, Ragette et al 2002). Each of these tests can be easily carried out at the bedside as part of the overall assessment of a patient presenting in respiratory failure. Strong use of the accessory respiratory muscles at rest, including the sternomastoid and the abdominal muscles, should raise the possibility that respiratory function may worsen during sleep.
In general, if there is awake hypercapnia then there will be substantial sleep-linked worsening of respiratory failure (Piper & Sullivan 1994a), although the converse does not necessarily hold true. Many subjects with awake CO2 within the normal range will have significant sleep-linked respiratory failure. The failure of clinicians to recognize this and intervene appropriately puts a patient at risk of developing daytime respiratory failure within the following 12–24 months (Ward et al 2005).
The limitations of daytime indices as predictors of nocturnal hypoventilation mean that more detailed sleep investigations may be required in order to accurately assess the severity and nature of the breathing disorder. While hypoventilation and desaturation are likely to occur predominantly in REM sleep, upper airway obstruction may also be present and needs to be identified and appropriately managed in order for NIV to be maximally effective. In patients with suspected nocturnal hypoventilation, monitoring of carbon dioxide is important looking at evening to morning changes in arterial values or continuous monitoring with transcutaneous measures (Ward et al 2005). Regardless of the investigations used, a high index of suspicion in patients with diagnoses known to be associated with nocturnal hypoventilation is warranted. In these individuals, baseline and serial testing of respiratory function should be performed with the view to identifying any deterioration early so that more specific investigation can be undertaken.
Conditions
Kyphoscoliosis
The final stages of severe kyphoscoliosis have been characterized by progressive respiratory failure associated with severe nocturnal hypoventilation (Ellis et al 1988). REM hypoventilation is probably caused by a combination of a very high work of breathing due to low chest wall compliance for a diaphragm that is at a significant mechanical disadvantage. In some patients sleep-disordered breathing is also complicated by upper airway obstruction. Mask ventilation is particularly suitable for these patients as other methods of assisted ventilation are very difficult. Tracheostomy can be problematic because of the loss of the extrathoracic trachea and the fitting of a cuirass is made exceptionally difficult by the chest wall deformity. Non-invasive ventilation can be readily achieved with a mask in this group despite the stiffness of the chest wall (Ellis et al 1988).
Cystic fibrosis
Although low-flow oxygen therapy has been the mainstay of treatment for patients with cystic fibrosis (CF) who develop respiratory failure, several reports have shown that, at least acutely during sleep, oxygen therapy can promote CO2 retention (Gozal 1997, Milross et al 2001). The beneficial effects of nocturnal non-invasive ventilation for patients with end-stage CF are recognized. Non-invasive ventilation has been shown to be of value during periods of acute deterioration, where marked pulmonary deterioration occurs despite maximum conventional therapy (Piper et al 1992). Use of mask ventilatory support in this setting can correct hypoxaemia without inducing additional CO2 retention. In addition, this technique may also be used to stabilize the patient in the short term while donor organs become available (Hodson et al 1991) or on a longer-term basis, allowing the patient to return home (Piper et al 1992). Although in initial reports volume preset machines were used, bilevel pressure devices are now being increasingly used with similar outcomes (Gozal 1997, Milross et al 2001).
Some patients report improved sputum clearance after initiation of nasal ventilatory support, possibly related to better tolerance of longer chest physiotherapy sessions (Piper et al 1992). Improved lung expansion and chest wall excursion while on the machine may also play a part. Studies have shown that use of nasal ventilatory support during chest physiotherapy was able to ameliorate adverse effects such as reduced respiratory muscle performance and oxygen desaturation (Fauroux et al 1999, Holland et al 2003).
Duchenne muscular dystrophy
Ventilatory support is often reluctantly prescribed for patients with progressive neuromuscular disease, owing to a perceived lack of quality of life for these patients. However, health professionals often underestimate quality of life in such patients. The use of long-term non-invasive ventilation has been shown to stabilize pulmonary function and prolong life expectancy while improving quality of life in patients with Duchenne muscular dystrophy (DMD) and awake hypercapnia (Simonds et al 1998). Non-invasive ventilation has also been initiated early in this disorder in an attempt to prevent the decline in lung function that occurs with increasing respiratory muscle weakness. However, no benefits from such a strategy could be identified (Raphael et al 1994). Therefore, NIV is an effective long-term therapy in this group of patients once nocturnal hypoventilation occurs, but may not have a prophylactic role.
Chronic obstructive pulmonary disease
Nocturnal nasal ventilation has been used effectively in selected patients with stable chronic obstructive pulmonary disease (COPD). However, this form of therapy is not tolerated as well as in other diagnostic groups and longer-term outcomes are not as favourable as in patients with neuromuscular and chest wall disorders (Simonds & Elliott 1995). Those patients most likely to benefit from nocturnal ventilatory support appear to be those with significant daytime hypercapnia, who have symptomatic sleep problems and in whom nocturnal hypercapnia can be successfully reduced by overnight ventilation. Meecham Jones et al (1995) reported a randomized crossover study of nasal pressure support ventilation plus oxygen therapy compared with domiciliary oxygen therapy alone in 18 hypercapnic patients with COPD. Improvements in daytime arterial blood gas tensions, overnight transcutaneous carbon dioxide (TcCO2), total sleep time and sleep efficiency were seen during non-invasive ventilation and oxygen therapy compared with oxygen therapy alone, suggesting that control of hypoventilation with non-invasive ventilation can be achieved. Importantly, these authors found that those who showed the greatest reduction in nocturnal hypercapnia with ventilation were likely to gain the greatest benefit from the treatment. However, a number of other randomized trials in this population have failed to find a clinically significant benefit of NIV (Casanova et al 2000, Clini et al 2002). These discrepant results may be due to patient selection or the way in which NIV was delivered and monitored. It would seem that patients with high daytime CO2, with a higher likelihood of nocturnal hypoventilation, are more likely to respond to this therapy than patients with near normal awake CO2 levels. In addition, data from Meecham Jones et al (1995) as well as uncontrolled trials (Windisch et al 2005) highlight the importance of using sufficiently high inspiratory pressures to control CO2 during sleep in order to achieve improvements in daytime ventilation and symptoms.
Motor neuron disease
Respiratory insufficiency usually occurs as a late manifestation of this disorder, when global peripheral and respiratory muscle weakness has occurred. However, in a small number of patients, presentation with hypercapnia, severe orthopnoea and sleep fragmentation may be seen. Although nasal ventilatory support has been shown to be effective in relieving these symptoms in this group, its use also raises some ethical and clinical concerns that need to be discussed with the patient and their caregiver (Polkey et al 1999). There has been reluctance to initiate such therapy for a condition that is known to be relatively rapidly progressive and where many will experience involvement of the bulbar muscles and swallowing difficulties (Meyer & Hill 1994). However, a recent randomized controlled trial has shown that NIV improves survival while maintaining and even improving quality of life in these patients (Bourke et al 2006). In those with severe bulbar impairment there was no survival benefit with NIV, but quality of life related to symptoms did improve.
Non-invasive ventilation appears to have a place as a management alternative in motivated patients with appropriate home supports, where established respiratory failure is present or where quality of life is impaired by sleep disruption or severe orthopnoea (Polkey et al 1999). However, before undertaking such therapy in this group, frank discussion with the patient and caregiver needs to occur. Potential benefits of NIV in palliating symptoms should be discussed as well as its limitations in the face of progressively worsening respiratory and general muscle strength and disability.
Obesity hypoventilation syndrome
Another group that responds rapidly and positively to non-invasive ventilation are those patients with obesity hypoventilation syndrome (Perez de Llano et al 2005, Piper & Sullivan 1994b). This syndrome is characterized by extreme obesity, excessive daytime sleepiness and severe derangement of awake blood gases. Patients frequently present grossly decompensated with right heart failure, lower limb oedema and hypercapnia. Use of non-invasive ventilatory support in these patients may result in improved awake blood gases and clinical condition within days of starting therapy, improving quality of life and daily function. The aim of therapy in these patients is to maintain upper airway stability while improving alveolar ventilation. Bilevel ventilatory support is usually required initially, particularly in those with severely deranged nocturnal and awake blood gases. However, after a short period of nocturnal ventilatory support, a proportion of patients can be transferred to the more simple CPAP therapy for longer-term domiciliary use (Perez de Llano et al 2005, Piper & Sullivan 1994b).
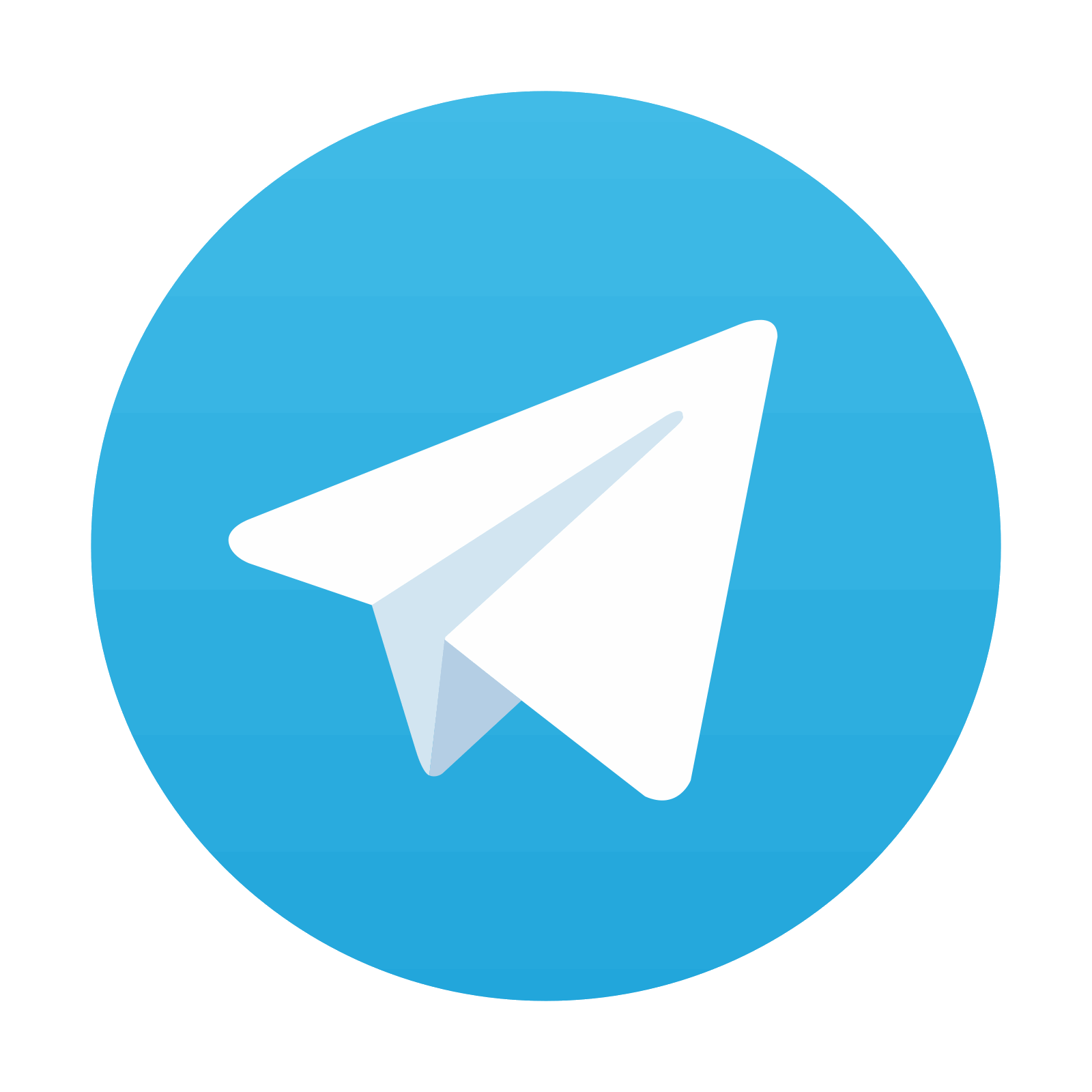
Stay updated, free articles. Join our Telegram channel
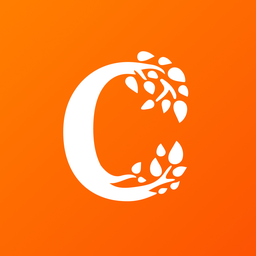
Full access? Get Clinical Tree
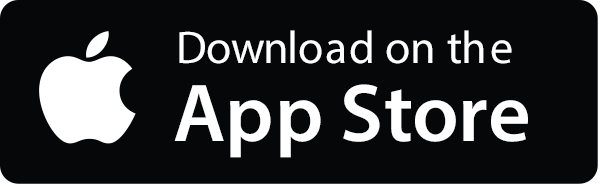
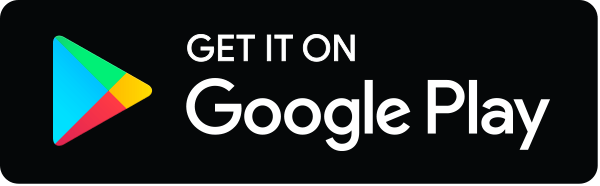