Background
Many implantable ventricular assist devices (VADs) have no direct measurement of pump output. The aim of this study was to test the hypothesis that quantitative contrast echocardiography can be used to measure VAD output.
Methods
Contrast-enhanced Doppler velocity-time integral (VTI) was measured in the VAD inlet and outlet cannulae. Doppler flow (Doppler Q = Doppler VTI × cannula area) was compared with measured flow ( Q ). A total of 130 flow measurements were made (at 6400 and 12,000 rpm).
Results
Doppler Q in the outflow and inflow cannulae showed an excellent correlation with measured Q (outlet Doppler Q = 1.0052 × Q + 0.048, R 2 = 0.9865; inlet Doppler Q = 1.5043 × Q + 0.003, R 2 = 0.9904), but inlet Doppler Q was 50% higher. Correcting for the flow profile of the conical inlet tube yielded excellent correlation (inlet Doppler Q = 1.0029 × Q + 0.002, R 2 = 0.9904).
Conclusion
Noninvasive Doppler flow techniques can be used to accurately measure VAD flow, but the flow profile in the cannula needs to be taken into account.
Similar to the native human heart, the performance of a ventricular assist device (VAD) depends on 3 factors: the inflow pressure, the pump’s pressure-flow performance characteristics, and the outflow load. In clinical settings, the inflow pressure, or preload component, is usually not known precisely and may be affected by factors extrinsic to the assisted ventricle, such as right-heart failure in patients with left-sided VADs. Likewise, the pump’s pressure-flow performance characteristics may be affected by occult intrinsic issues, such as thrombus within the device, or other mechanical derangements. Lastly, the outflow load parameter is usually qualitatively estimated using the systemic blood pressure, because a more accurate measure of arterial resistance requires full invasive instrumentation.
Precise knowledge of VAD output would be beneficial in the management of individual patient hemodynamics and in the determination of appropriate VAD settings. Unfortunately, many of the currently available implantable VADs have no direct measurement of pump output. Instead, these devices use calculations based on indirect measures, such as power consumed at a given RPM or VAD rate and stroke volume, to estimate output. These indirect measures of VAD output are usually reasonably accurate when the device is operating normally and the patient is hemodynamically stable. Unfortunately, the indirect measures of VAD output may be inaccurate in some device failure conditions or when extrinsic factors lead to hemodynamic instability. It is in these conditions when an independent quantitative measure of VAD performance would be the most clinically useful.
In this protocol, a continuous-flow rotary pump was tested in an in vitro flow system designed to simulate the hemodynamics of the human circulation to test the hypothesis that quantitative contrast echocardiography can be used to accurately measure the output of VADs.
Methods
Theoretical Model
The theoretical model tested in this protocol was that the product of a pulsed-wave spectral Doppler velocity-time integral (VTI) from the middle of a flow tube and the flow tube’s cross-sectional area would equal the flow volume (Doppler Q ) for the period of time integrated:
Doppler Q ( cm 3 / min ) = VTI ( cm ) × area ( cm 2 ) × 60 ( s / min ) integral duration ( s ) .
This technique is well known and used daily in clinical settings to calculate the left ventricular stroke volume and cardiac output, in which the “flow tube” of choice is usually the left ventricular outflow tract and the time period of integration is a single systolic ejection period. The method was applied here to calculate the VAD minute flow rate using either the VAD inflow or outflow cannula as the “flow tube.” Rotary impeller-style VADs produce continuous flow and do not have a clearly defined “stroke volume.” Rather, the Doppler minute flow rate is calculated directly by normalizing traced spectral VTI segment to sixty seconds and then multiplying by the cannula cross-sectional area.
Flow Circuit
A continuous-flow rotary pump (HeartMate II; Thoratec Corporation, Pleasanton, CA) was tested in a flow system consisting of plastic tubing and pressurized reservoirs designed to simulate the left side of the human circulation ( Figure 1 ). The tubing and devices on the inflow and outflow sides of the HeartMate II are designated as “venous” and “arterial,” respectively. A sealed rigid plastic chamber functioned as a venous reservoir and a second similarly sealed rigid plastic chamber functioned as the arterial reservoir. Both of these chambers were roughly half filled with distilled water. The trapped air spaces above the water in the reservoirs were individually pressurized using a hand pump and pressure gauge. The two reservoirs were connected by a single plastic tube so that arterial volume could be returned to the venous reservoir. The connector tube between the reservoirs was restrictive, so that an arterial-venous pressure differential was maintained. The connector tube’s resistance was adjusted as needed on the basis of the flow rates so that steady-state reservoir levels were maintained.

The single outlet of the venous reservoir fed into a modified pneumatically driven pulsatile VAD (PVAD) (Thoratec LVAS; Thoratec Corporation). This device was in the circuit to provide pulsatile inflow to the HeartMate II when it was active, and it functioned as a passive conduit when inactive. The PVAD was modified from the standard clinical unit by having its outlet valve removed. This was designed so that the PVAD added only a low-intensity pressure pulse to the system (fully regurgitant), while still allowing for continuous flow though the PVAD. The flow exiting the PVAD was directed toward the HeartMate II, but a pressure-relief circuit was also present that allowed excess PVAD output to return to the arterial reservoir. A check valve was present in this pressure-relief circuit to prevent arterial flow (pressure) from entering the venous side of the system.
The HeartMate II was mounted in a specially fabricated plastic chamber designed to simulate a standard apical left ventricular implant. The plastic chamber allowed for pressure monitoring and was topped with a Mylar window, which allow for axial pulsed-wave Doppler and two-dimensional echocardiographic imaging of the HeartMate II inflow cannula. A pressure monitoring port (Hewlett-Packard Corporation, Andover, MA) and venous access port for contrast injections were incorporated into the inlet imaging chamber. A standard clinical Dacron woven graft was connected to the HeartMate II exit outflow port. The outflow graft was positioned in a water tank outfitted with acoustic absorbent material, which allowed for high-quality pulsed-wave Doppler and two-dimensional echocardiographic imaging of the tube. The arterial circuit was completed by monitoring the flow with a clamp-on ultrasonic flow probe (Q Meter; Transonic Systems, Ithaca, NY) and for pressure, before it was returned to the arterial reservoir. A resistive clamp was also on the return line, which allowed for adjustment of the arterial resistance during pump runs. The resistive clamp was specially built to provide precision partial occlusion of the tubing and to be unaffected by the pulsing effects of the PVAD.
Ultrasound Imaging
Two-dimensional and pulsed-wave Doppler ultrasonic imaging was performed simultaneously of the inlet and outlet cannulae using twin Acuson Sequoias (Siemens Medical Solutions USA, Inc, Mountain View, CA). Two-dimensional imaging was used to measure the internal diameters of the inlet and outflow cannulae, and pulsed-wave Doppler ultrasound was used to measure the velocity profile in the center of the flow tube. Inlet cannula imaging was performed using a V5M multiplane transesophageal echocardiographic probe, which was secured to the Mylar imaging window of the special imaging chamber described above. A multiplane transesophageal echocardiographic probe was used because this is the device that would likely be used for inflow cannula imaging in clinical settings. The pulsed-wave Doppler range gate was set to 5 mm length and was centered within the inflow cannula 1.0 cm from the orifice and 110 cm from the transducer face. Outlet cannula imaging was performed using a 6L3c vascular transducer held in a rigid clamp at an angle to the cannula so that an angle correction factor of only 30° needed to be used. This degree of transducer tilt and Doppler angle correction would be typical for in vivo imaging of the outflow cannula. The pulsed-wave Doppler range gate was centered in the outflow cannula flow tube approximately 3.9 cm from the transducer face and set to 5 mm length. The imaging parameters for both systems were otherwise similar, with a pulsed-wave Doppler frequency of 3.5 MHz and an imaging frequency 6.0 MHz. All two-dimensional and Doppler traces were digitally recorded in Digital Imaging and Communications in Medicine format and analyzed for VTI offline using the built-in analysis software included with the KinetDx picture archiving and communication system (Siemens Medical Solutions USA, Inc). In addition, Doppler audio signals were recorded on high-fidelity S-VHS videotape and analyzed offline using custom software that has been previously described and validated. Briefly, the audio Doppler signals were digitized at 24 kHz and analyzed with quantitative spectral analysis to determine the power peak frequency (modal velocity) and spectral peak frequency (corresponding to the maximum velocity measured on the video spectral traces).
Contrast Agent
Definity echocardiographic contrast (Bristol-Meyers Squibb, North Billerica, MA) was used in very dilute quantities to enhance the Doppler signal and improve the resolution of the spectral peak velocity. Very small boluses of Definity were introduced into the flow system, and 2 minutes were allowed for the concentration in the closed system to equilibrate. The dose of contrast was chosen to maximize Doppler enhancement without attenuation noted on two-dimensional or color Doppler imaging, and additional doses were added to the system as needed to maintain Doppler quality. Qualitative dosing of this type has been well established in clinical settings.
Flow Protocol
The HeartMate II pump speed was adjusted from 6400 to 12,000 rpm in 13 steps (6400, 7200, and then 8000 to 12,000 rpm in 400-rpm steps). At each pump speed, a series of 5 afterload resistances were used, ranging from no additional resistance above that of the flow tubing to complete occlusion. To assess the effects of pulsatile compared with nonpulsatile inflow pressures on VAD performance, the HeartMate II flow was measured with the PVAD active and inactive at each pump speed setting. When active, the PVAD was set to a heart rate of 80 beats/min, a positive drive pressure of 100 mm Hg, and 0 mm Hg of vacuum pressure.
Image Analysis
The cross-sectional areas of the inflow and outflow flow tubes were calculated from their two-dimensional imaging–measured diameters using the equation for the area of a circle. The spectral peak velocity of the pulsed-wave Doppler spectrum was hand traced over a period of 3 seconds to calculate the VTI ( Figure 2 ). The minute flow rate was obtained from product of 20 times the 3-second pulsed-wave Doppler VTI and the cross-sectional area of the flow tube. The calculated inflow and outflow minute flow rates were compared with the flow meter–measured flow rates using linear regression. All inflow and outflow Doppler VTI measurements with subsequent flow calculations were made by two independent observers blinded to each other’s results. Interobserver variability analysis was performed on these paired measurements and demonstrated excellent correlation (linear regression fit, y = 1.0094 x + 0.0082, R 2 = 0.99295), and Bland-Altman analysis showed no significant bias.

Flow Contour Assessment
A series of 7 pulsed-wave Doppler samples were made from edge to edge across the inflow and outflow cannulae to measure the flow profile and determine if a central-tubing Doppler sample position is adequate to accurately quantify the volumetric flow rate. At each position, the audio Doppler signal was analyzed for power-peak and spectral-peak frequencies (velocities). The power-peak frequency represents the Doppler modal velocity and is largely independent of the system gain and settings. The spectral-peak frequency represents the spectral Doppler outer envelope, that is usually traced clinically from Doppler spectral video images. Numerical analysis was used to fit the power-peak and spectral-peak velocities to the radial distance across the flow tube. The resultant transfer functions were used to correct the inflow cannula Doppler VTI measurements.
Statistical Analysis
Statistical data are presented as mean ± SD. Linear regression was used to test the relationship between the Doppler VTI–measured flow and flow meter–measured flow.
Results
A total of 130 flow measurements in both inflow and outflow cannulae were obtained from a combination of 13 HeartMate II pump speeds, 5 different afterload conditions, and pulsatile versus nonpulsatile inflow variation. The spectrum of hemodynamic conditions thus created mimic that seen in typical clinical settings ( Table 1 ). Linear regression showed an excellent correlation between the echocardiography-calculated flow rates in the inflow and outflow cannulae with the measured flow rates ( Figure 3 ). Doppler-calculated flow in the outflow cannula showed an identity relationship with measured flow with no offset (outlet Doppler Q = 1.0052 × Q + 0.048; R 2 = 0.9865; slope confidence interval, 0.985 to 1.025; intercept confidence interval, −0.053 to 0.063). Inlet cannula Doppler-calculated flow also showed an excellent linear relationship with measured flow, but with a 50% offset (inlet Doppler Q = 1.5043 × Q + 0.003, R 2 = 0.9904). The results in both the inlet and outlet cannulae were independent of whether the input flow to the HeartMate II had a pulsatile waveform or was linear.
Parameter | Mean ± SD | Minimum | Maximum |
---|---|---|---|
Set rotational rate (rpm) | 9515 ± 1518 | 6400 | 12,000 |
Measured rotational rate (rpm) | 9512 ± 1518 | 6,340 | 11,995 |
Power (W) | 5.1 ± 1.7 | 2.0 | 8.8 |
Δ P (mm Hg) | 82 ± 34 | 21 | 173 |
Flow estimator (L/min) | 2.5 ± 0.5 | 1.6 | 3.8 |
Measured Q (L/min) | 2.34 ± 1.73 | −0.25 | 6.01 |
Inflow Q (L/min) | 3.51 ± 2.61 | −0.43 | 8.63 |
Outflow Q (L/min) | 2.35 ± 1.75 | 0.00 | 5.94 |
Corrected inflow Q (L/min) | 2.34 ± 1.74 | −0.29 | 5.76 |

The results of the flow contour assessment in the inflow cannula using the audio Doppler analysis showed a nonlinear relationship between flow velocity and radial position ( Figure 4 ). The spectral-peak flow velocity (open circles) was uniform and showed no relationship with radial position within the inlet cannula ( y = −0.0006 x + 0.3401, R 2 = 0.18), whereas the power-peak flow velocity (modal velocity, filled circles) showed a complex relationship with radial distance ( y = 0.428[1 − ( x / R ) 4 ], R 2 = 0.979). Integrating the correction factor over width of the inflow cannula resulted in a correction factor of two thirds, meaning that the corrected mean flow VTI was 66% of that measured in the middle of the inlet cannula. Applying this correction factor to the inlet Doppler VTI measurements yielded excellent correlation with measured flow (inlet Doppler Q = 1.0029 × Q + 0.002; R 2 = 0.9904; slope confidence interval, 0.986 to 1.019; intercept confidence interval, −0.046 to 0.050). As the correction factor is linear, the shape of the curve is the same as that shown in Figure 3 ; only the slope and intercept are changed.

The flow contour analysis in the outflow cannula showed only a weakly negative relationship with imaging depth for spectral-peak flow velocity ( y = −0.0142 x + 0.4731, R 2 = 0.9757), whereas the power-peak flow velocity was essentially uniform across the flow tube, showing a nonsignificant relationship with imaging depth ( y = −0.067 x + 0.3237, R 2 = 0.4059; Figure 5 ).

Results
A total of 130 flow measurements in both inflow and outflow cannulae were obtained from a combination of 13 HeartMate II pump speeds, 5 different afterload conditions, and pulsatile versus nonpulsatile inflow variation. The spectrum of hemodynamic conditions thus created mimic that seen in typical clinical settings ( Table 1 ). Linear regression showed an excellent correlation between the echocardiography-calculated flow rates in the inflow and outflow cannulae with the measured flow rates ( Figure 3 ). Doppler-calculated flow in the outflow cannula showed an identity relationship with measured flow with no offset (outlet Doppler Q = 1.0052 × Q + 0.048; R 2 = 0.9865; slope confidence interval, 0.985 to 1.025; intercept confidence interval, −0.053 to 0.063). Inlet cannula Doppler-calculated flow also showed an excellent linear relationship with measured flow, but with a 50% offset (inlet Doppler Q = 1.5043 × Q + 0.003, R 2 = 0.9904). The results in both the inlet and outlet cannulae were independent of whether the input flow to the HeartMate II had a pulsatile waveform or was linear.
Parameter | Mean ± SD | Minimum | Maximum |
---|---|---|---|
Set rotational rate (rpm) | 9515 ± 1518 | 6400 | 12,000 |
Measured rotational rate (rpm) | 9512 ± 1518 | 6,340 | 11,995 |
Power (W) | 5.1 ± 1.7 | 2.0 | 8.8 |
Δ P (mm Hg) | 82 ± 34 | 21 | 173 |
Flow estimator (L/min) | 2.5 ± 0.5 | 1.6 | 3.8 |
Measured Q (L/min) | 2.34 ± 1.73 | −0.25 | 6.01 |
Inflow Q (L/min) | 3.51 ± 2.61 | −0.43 | 8.63 |
Outflow Q (L/min) | 2.35 ± 1.75 | 0.00 | 5.94 |
Corrected inflow Q (L/min) | 2.34 ± 1.74 | −0.29 | 5.76 |
The results of the flow contour assessment in the inflow cannula using the audio Doppler analysis showed a nonlinear relationship between flow velocity and radial position ( Figure 4 ). The spectral-peak flow velocity (open circles) was uniform and showed no relationship with radial position within the inlet cannula ( y = −0.0006 x + 0.3401, R 2 = 0.18), whereas the power-peak flow velocity (modal velocity, filled circles) showed a complex relationship with radial distance ( y = 0.428[1 − ( x / R ) 4 ], R 2 = 0.979). Integrating the correction factor over width of the inflow cannula resulted in a correction factor of two thirds, meaning that the corrected mean flow VTI was 66% of that measured in the middle of the inlet cannula. Applying this correction factor to the inlet Doppler VTI measurements yielded excellent correlation with measured flow (inlet Doppler Q = 1.0029 × Q + 0.002; R 2 = 0.9904; slope confidence interval, 0.986 to 1.019; intercept confidence interval, −0.046 to 0.050). As the correction factor is linear, the shape of the curve is the same as that shown in Figure 3 ; only the slope and intercept are changed.
The flow contour analysis in the outflow cannula showed only a weakly negative relationship with imaging depth for spectral-peak flow velocity ( y = −0.0142 x + 0.4731, R 2 = 0.9757), whereas the power-peak flow velocity was essentially uniform across the flow tube, showing a nonsignificant relationship with imaging depth ( y = −0.067 x + 0.3237, R 2 = 0.4059; Figure 5 ).
Discussion
Most currently available totally implantable VADs do not have built-in methods for the accurate quantification of VAD flow. Pulsatile pumps, such as the Thoratec IVAD and HeartMate XVE rely on mechanical constants to estimate flow. In the case of the Thoratec IVAD, the volume of air used to drive the pump is assumed to equal the volume of blood pumped. However, air is a compressible gas, so the volume of gas used to drive the pump might not always be equivalent to the volume of blood pumped. In the case of the HeartMate XVE a mechanical pressure plate is electrically driven, and the position of the pressure plate is used to calculate the stroke volume. Unfortunately, this calculation might be incorrect if there is a defect in the blood bladder or if there is a thrombus or other obstruction in the bladder or tubing.
Like other rotary pumps, the HeartMate II is controlled primarily by adjusting the rotational rate. The HeartMate II controller also provides a display of the power consumed, pulsatility index, and estimated flow rate. The flow estimator within the HeartMate II uses a proprietary formula to calculate VAD output based on the rotational rate, pulsatility index, and power consumed. The flow estimator is considered by the manufacturer to be reliable over only a relatively narrow, but clinically relevant, range of flow rates. The flow estimator was not tested directly in this protocol for 3 reasons: (1) not all rotary pumps have flow estimators, so the results would not be widely applicable; (2) the range of flow rates tested exceeded the design parameters of the flow estimator; and (3) the fluid pumped (water) has a different viscosity compared with blood, which would alter the estimated flow results.
The need for an accurate bedside method for the measurement of VAD flow cannot be underestimated. It is not uncommon that the hemodynamics of VAD patients cannot be entirely explained on the basis of standard clinical criteria, and in these circumstances, an independent measure of VAD performance is needed. This clinical situation most commonly arises when a patient presents with symptoms of low output and routine echocardiography fails to reveal a suspect cause (such as large pericardial effusion, inflow or outflow cannula obstruction, severe right-heart failure, etc). The clinician may be faced with VAD failure as a diagnosis of exclusion, and this may subject the patient to a VAD replacement procedure, which may or may not be needed. In addition, an accurate method for bedside measurement of VAD flow would allow the optimization of VAD settings. This is usually done using clinical parameters of output (blood pressure, heart rate, kidney function, patient symptoms, etc) combined with echocardiographic measures of cardiac performance (right-heart and left-heart size and function and the presence or absence of aortic valve opening). Although these methods are useful, they are only surrogate measures of VAD output. Combining these clinical measures with quantification of VAD flow allows for the optimal setting of the VAD parameters. We routinely perform “ramp testing” on our clinical VAD patients, which is a sequential assessment of VAD flow using the contrast Doppler method at different VAD rotational rate settings. The results of ramp testing are not only helpful in diagnosing VAD dysfunction but are also useful in determining the optimal VAD settings, even when a patient is clinically stable. We have found that the ramp test can be performed both with transesophageal echocardiographic imaging of the outflow cannula (usually the 90° transesophageal view of the tubular aorta) and also with transthoracic echocardiography. The latter is usually best performed by imaging the mid portion of the outflow cannula using a vascular linear array probe in either the second or third right intercostal space.
Doppler velocity and VTI measurements are almost always performed on the spectral Doppler traces produced by the echocardiographic machine. Although not widely appreciated, 3 pieces of information are contained in the Doppler spectral trace: (1) the intensity of the ultrasound backscatter, (2) the spectral-peak velocity, and (3) the power-peak velocity. The intensity of the ultrasound backscatter is represented by the intensity of the spectral trace, which is in part dependent on the Doppler gain and dynamic range setting of the spectral image. The spectral-peak velocity is the visible white-black interface that defines the outer envelope of the spectral Doppler trace and so is affected by the Doppler settings (including gain and dynamic range). The power-peak velocity is the modal or mean velocity of the flow system, and is seen as the brightest spot in the spectral Doppler trace. Figure 6 shows that the spectral peak velocities are similar in the Doppler trace created from the middle of the inflow cannula compared with the edge, but there is clear spectral broadening as the Doppler sample volume is moved from the center to the edge of the inlet cannula. This indicates that the mean velocity has clearly shifted with the position of the sample volume, but the power-peak or modal velocity is not easily discernible from the spectral trace. Using Fourier analysis on the audio Doppler signal, the shift in the power-peak velocity can be easily seen. This technique was used to define the flow profile in the inlet cannula of the HeartMate II device. The correction factor was shown to vary with the fourth power of the cannula radius, but simplified to a correction factor of two thirds for VTI measurements made in the center of the flow tube (see Appendix A for derivation). Applying this correction factor resulted in excellent accuracy and correlation with measured flow in the conical inlet cannula.
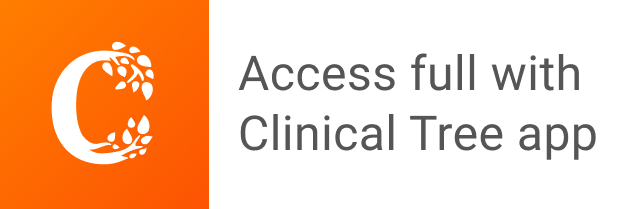