Fig. 21.1
Diagrams showing the basic principles of 2D and 3D time of flight (TOF) angiography. A tortuous artery traversing through tissue is shown; arrows show the direction of arterial flow and brightness corresponds to the signal observed at MR imaging. Horizontal red lines demarcate slices and slabs for 2D and 3D TOF MRA, respectively. Stationary tissue experiences repeated RF pulses, which saturate its magnetization and suppress its appearance. Blood vessels experience inflow enhancement, which render them hyperintense. The degree of arterial hyperintensity diminishes as arterial spins flow farther into the slab and become saturated; this effect is best appreciated with single-slab 3D TOF MRA (third column). With 2D TOF MRA (first column), horizontally directed vessel segments are partially saturated and vascular contrast is diminished (*); the use of tracking venous saturation immediately above the imaging slice suppresses arterial segments containing retrograde flow (†, second column). Saturation of inflowing arterial magnetization during 3D TOF MRA can be lessened with the acquisition of multiple overlapping or adjacent thin slabs (fourth column)
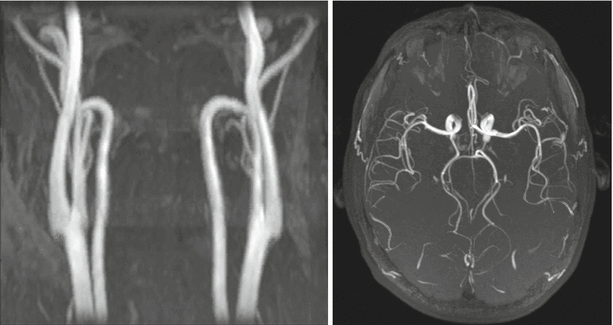
Fig. 21.2
3D time of flight (TOF) MR angiograms obtained in the carotid arteries (left panel; coronal maximum intensity projection) and intracranial arteries (right panel; transversal maximum intensity projection) at 1.5 Tesla
With TOF MRA, arterial signal and contrast with respect to surrounding background tissue depends on several factors including the longitudinal relaxation times (T1s) of arterial blood and prevailing background tissue (which depend on the magnetic field strength), the effective thickness of the imaging slice or slab which corresponds to the distance arterial blood must travel within the slab, the interecho spacing, the imaging flip angle, and the velocity of inflowing arterial blood. For a fixed interecho spacing and slab thickness, the use of larger flip angles with 3D TOF MRA improve background suppression but reduce the conspicuity of vessels located deeper within the slab.
Compared to 3D TOF MRA, 2D TOF imaging reduces inflow requirements for achieving optimal arterial opacification. Accordingly, 2D TOF MRA protocols typically use larger flip angles and achieve better arterial-to-background contrast than 3D TOF MRA methods. On the other hand, 3D TOF MRA can provide higher resolution in the slice direction than 2D TOF MRA. In either implementation, placement of the imaging slice (for 2D TOF) or slab (for 3D TOF) perpendicular to the blood vessels minimizes inflow requirements and optimizes vascular signal.
Suppression of non-vascular background signal during TOF imaging is achieved through the repeated application of imaging RF pulses. Compared with TOF imaging at 1.5 T, 3.0 T imaging provides improved background tissue suppression due to longer T1 relaxation times. Additional suppression of background tissue with significant macromolecular content (such as muscle or gray matter) is possible by applying magnetization transfer RF pulses within the echo train. The impact of 3 T imaging and magnetization transfer RF pulses on the arterial contrast of 3D TOF MRA is shown in Fig. 21.3.
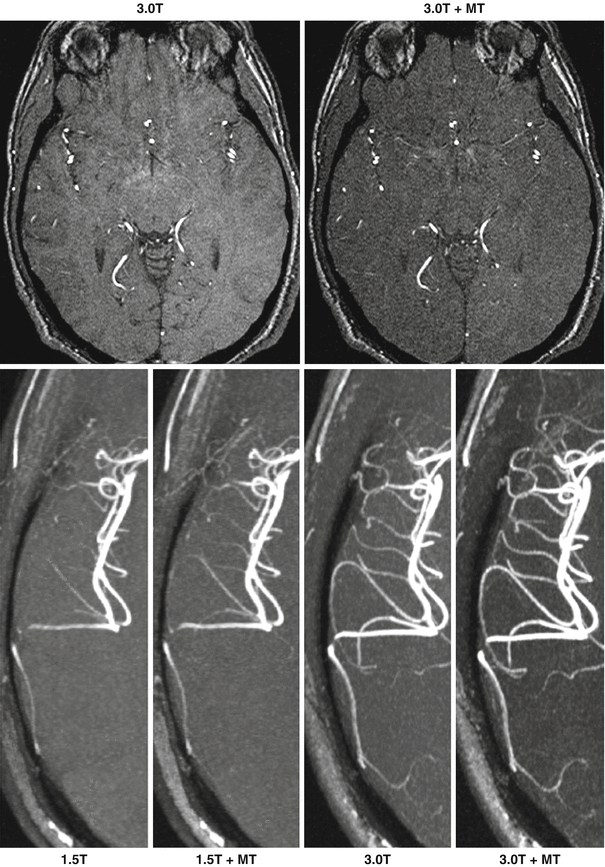
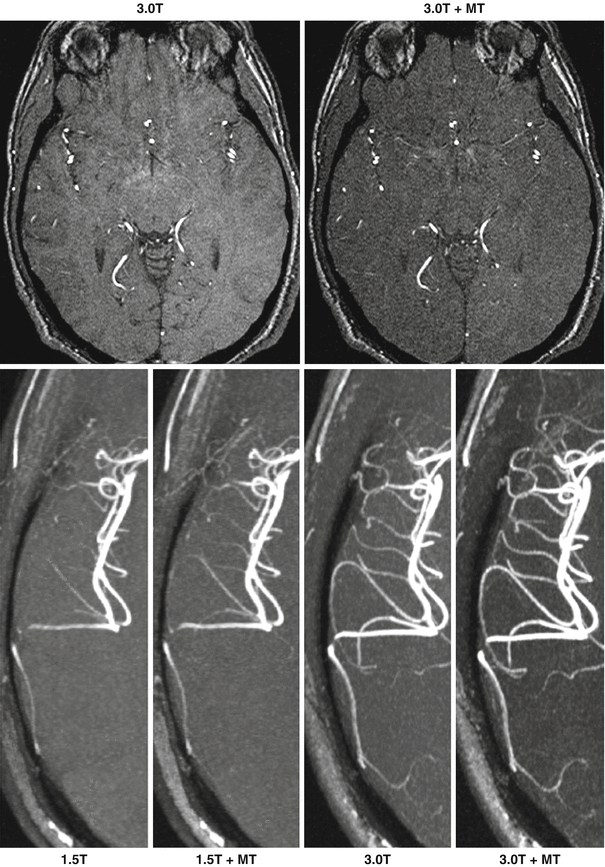
Fig. 21.3
Impact of magnetization transfer contrast (MT) and magnetic field strength on 3D TOF MRA of the intracranial arteries. Top panel: axial source images show that magnetization transfer improves the suppression of brain parenchyma. Bottom panel: axial maximum intensity projection (MIP) images show the improved arterial display and background tissue suppression obtained at higher field strength, and the improvements associated with magnetization transfer contrast at 1.5 T and 3.0 T (Reprinted from Thomas et al. [21] with permission from John Wiley and Sons)
Although traditional TOF methods use single-echo readouts, some studies in the brain have explored dual-echo TOF techniques to improve arterial-to-background contrast or provide additional venographic information. By acquiring a TOF angiogram with the first gradient echo and dephasing the appearance of flowing spin in the second echo, the appearance of background tissue can be suppressed by subtracting the image derived from the second echo from that of the first echo. Alternatively, the second echo can be used for dark-blood venography (bright-blood venography with inversion of the grayscale) because it is sensitive to shortened T2* from deoxygenated blood in cerebral veins.
Advantages, Limitations and Technical Considerations
Advantages of TOF MRA include widespread availability on commercial MRI systems at 1.5 T as well as 3.0 T, and good opacification of arteries containing rapid or continuous flow, such as the carotid and intracranial arteries. Drawbacks of TOF MRA include relatively slow acquisition times which prohibit routine screening of long vascular lengths, and imaging artifacts. Artifacts encountered with TOF MRA include arterial signal degradation in vessels containing turbulent, slow, or retrograde flow (which can be exacerbated by tracking venous saturation RF pulses), tortuous vessels, artifacts due to motion, and signal loss in vascular segments with severe stenosis.
Applications of Time of Flight MRA
Time of flight MRA is most commonly applied to neurovascular imaging where a relatively small anatomical coverage is required, and where flow is fairly rapid and continuous and there is little physiological motion. Multiple overlapping thin slab acquisition (MOTSA) 3D TOF MRA is used to evaluate the intracranial vessels, combining some of the benefits of 3D TOF MRA while minimizing flow saturation effects. With the use of parallel imaging, acquisition times of the order of 3–4 min can be achieved.
Common neurovascular applications include imaging for steno-occlusive intracerebral disease, in the setting of suspected acute cerebral infarction/ischemia. TOF MRA can be performed at the same examination as diffusion and perfusion weighted imaging to identify infarct and ischemic penumbra. Another common application is in the assessment of cerebral aneurysms, including screening patients predisposed to aneurysm formation, assessment of known aneurysms and post-treatment follow up, particularly for previous endovascular coil occlusion. Improved performance at 3.0 T compared to 1.5 T has been reported, as higher spatial resolution is achievable. Susceptibility artifact related to previously surgically clipped aneurysms may preclude identification of small volume recanalization. TOF MRA performance is poorer in acute non-traumatic subarachnoid hemorrhage, due to challenges of patient clinical status and presence of blood in the subarachnoid space.
Other potential applications include screening for occult vascular malformations, or evaluation of the extracranial carotid and vertebral arteries for dissection or steno-occlusive disease as an alternative or adjunct examination where contra-indications to CT or MR with contrast exist.
2D TOF MR venography is also used in the assessment of dural venous sinus thrombosis. Similar to in arterial ischemia, a comprehensive MR examination can be performed that enables direct visualization of thrombus, as well as evaluation of potential complications including venous infarct or intracerebral hemorrhage.
Apart from anatomic assessment, TOF MRA can also be applied for determining flow directionality, which may be helpful in suspected subclavian steal, where reversal of flow in the ipsilateral vertebral artery is observed, or in body imaging, including assessment of directionality of portal vein flow in portal hypertension.
One potential pitfall of TOF MRA is that any tissues with short T1 relaxation times (including intracellular and extracellular methemoglobin) may fail to be suppressed by excitation pulses and be mistaken for flow. As such, intracerebral hematoma may for example be mistaken for a giant cerebral aneurysm, or may mask an underlying vascular malformation if images are not interpreted in conjunction with other sequences, where lack of flow at the same location should enable differentiation. Also, turbulent, slow or in-plane flow can lead to overestimation of stenosis due to saturation effects.
Subtractive Arterial Spin Labeled Angiography
Overview and Physical Principles
Arterial spin labeling (ASL) is an approach for nonenhanced MR angiography that acquires two image sets which are later subtracted. The image sets differ only in the magnetization states of arterial spins. The process by which this difference in arterial magnetization is imparted is referred to as ‘labeling.’ Subtraction of the image sets displays the labeled arteries and provides complete suppression of background signal.
The first reports of ASL for nonenhanced MR angiography were made in the mid and late 1980s [3–5]. Early implementations of ASL for MR angiography used spin-echo and gradient-echo readouts, but most modern implementations now utilize readouts that are faster and more SNR-efficient. Two popular readouts meeting these criteria are balanced steady-state free precession imaging (bSSFP) and fast spin-echo (FSE) imaging.
As will be explained, labeling of arterial magnetization in ASL MRA can be performed in two ways. Arterial spin labeling can be achieved by either applying RF pulses to alter arterial magnetization, or relying on flow-related dephasing made possible by magnetic field gradients.
Arterial Spin Labeling with Radiofrequency Pulses
Labeling of arterial spins with inversion RF pulses is often considered the most traditional form of arterial spin labeling. This variation of arterial spin labeling is often referred to as ‘pulsed’ ASL. In its most basic form, this method consists of applying an inversion recovery RF pulse, typically of adiabatic type, to arterial spins flowing into the target vasculature, followed by an inversion delay time (TI), and an imaging readout. The difference of this data set and one acquired without the inversion RF pulse selectively displays the labeled arterial spins on a background of no signal. This labeling approach is often referred to as “Signal Targeting with Alternating inversion Recovery” (STAR) [6]. An illustration of STAR ASL MRA is shown in Fig. 21.4a.
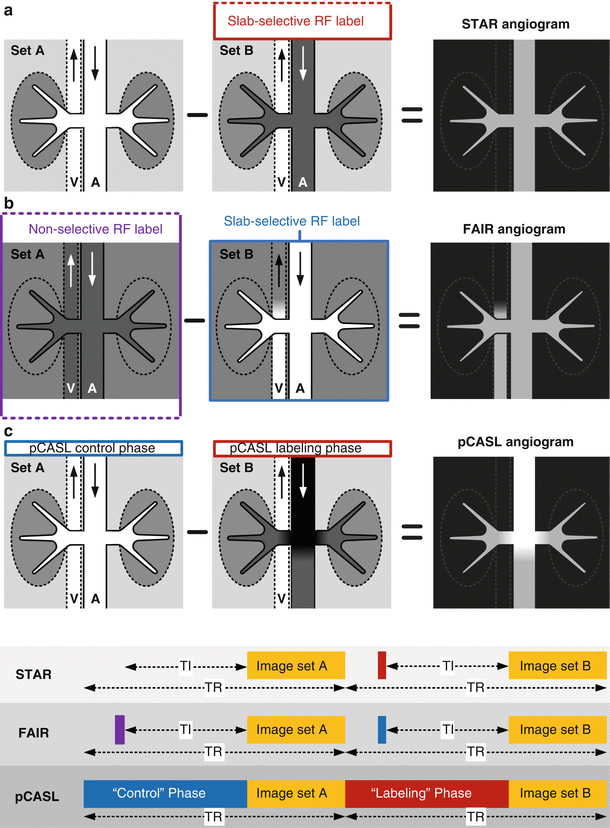
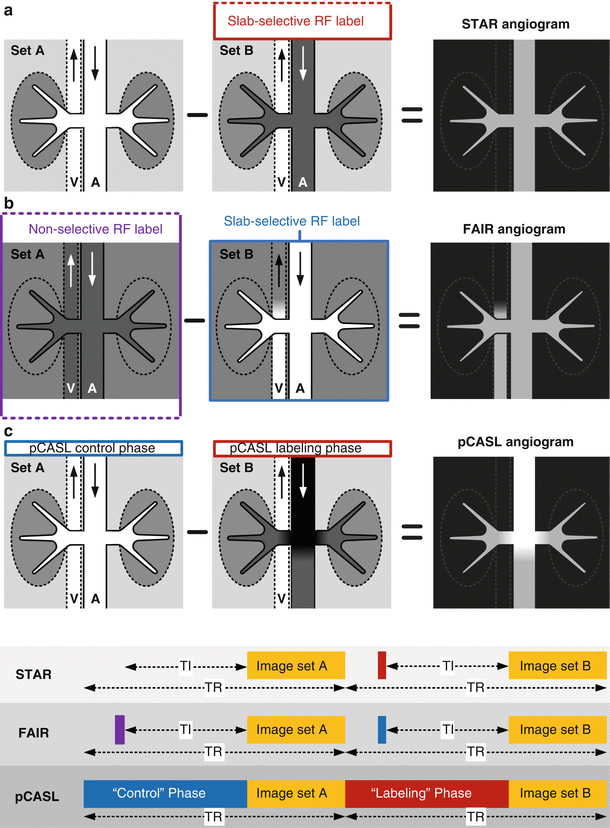
Fig. 21.4
Illustration of (a) STAR, (b) FAIR, and (c) pCASL techniques for nonenhanced MRA. For each technique, a cross-sectional view of the imaging slab is shown containing a cartoon of the aorta (A) and inferior vena cava (V). STAR and FAIR utilize either selective or non-selective inversion RF pulses for spin labeling. During pCASL MRA, RF labeling is applied to a thin plane upstream of the arterial anatomy under interrogation. RF energy applied during the pCASL “control” phase is effectively inert and leaves inflowing arterial blood largely unperturbed. RF energy applied during the pCASL “labeling” phase inverts inflowing arterial magnetization. With all techniques, subtraction of the two acquired data sets produces a MR angiogram with excellent background suppression. Sequence timing diagrams are shown at the bottom. TR repetition time, TI inversion time
Another approach for pulsed arterial spin labeling is “Flow Alternating Inversion Recovery” (FAIR) (Fig. 21.4b) [7]. This approach applies a slice selective inversion RF pulse to the target vasculature in one set and a non-selective inversion RF pulse to the second data set. Subtraction of the data sets displays vascular spins flowing into the target region. Compared with STAR, FAIR ASL may, depending on venous orientation and flow rate, display venous flow into the target region which can be undesirable. As compared to STAR, the FAIR approach better equalizes magnetization transfer effects between the two acquired data sets, which can improve the suppression of background tissue. This magnetization transfer effect, however, is usually not a concern with nonenhanced ASL MRA because of its small magnitude compared to the large signal within arteries.
Unlike pulsed arterial spin labeling, which applies short RF inversion pulses at a fixed TI time before imaging, another class of ASL techniques apply a continuous stream of RF energy for spin labeling. These methods are referred to as “continuous” or “pseudo-continuous”. Whereas continuous arterial spin labeling requires the use of a separate labeling RF coil which limits widespread use in the clinical setting, pseudo-continuous arterial spin labeling (also referred to as “pulsed-continuous” arterial spin labeling) can be applied without additional hardware requirements [8]. An implementation of pseudo-continuous ASL (pCASL) is shown in Fig. 21.4c. Compared to pulsed techniques, pCASL can provide larger arterial signal to noise ratio (SNR) in regions near the pseudo-continuous labeling plane where short effective inversion times are realized. Drawbacks of pCASL include increased RF energy deposition compared with pulsed approaches, and increased sensitivity to off-resonance as well as flow velocity which can reduce labeling efficiency and arterial SNR. Example MR angiograms obtained with pulsed and pseudo-continuous ASL are shown in Fig. 21.5.
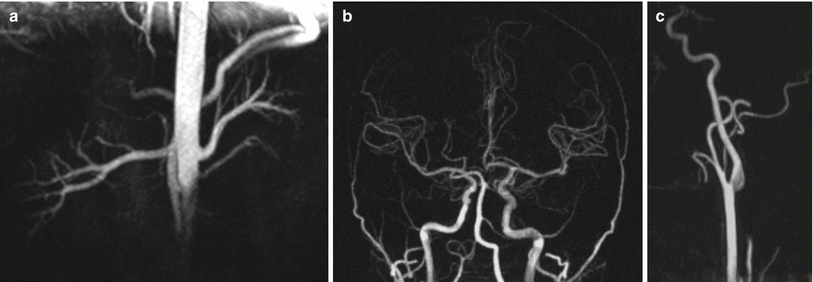
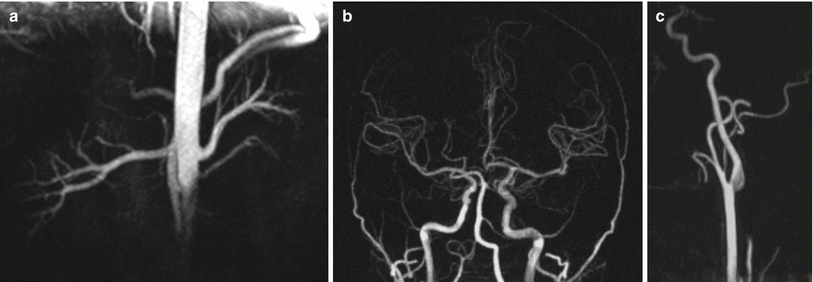
Fig. 21.5
Examples of arterial spin labeled MR angiography acquired using a bSSFP readout at 1.5 T. (a) Nonenhanced renal MR angiogram obtained with STAR arterial spin labeling and respiratory gating. (b) Intracranial and (c) extracranial carotid MR angiograms obtained with combined pseudo-continuous and pulsed arterial spin labeling. All images are maximum intensity projections
Arterial Spin Labeling with Gradient Dephasing
Another means for labeling arterial spins during ASL is based on differential flow between systole and diastole in vessels containing pulsatile flow. This approach, originally referred to as gated subtraction angiography [3, 5], subtracts data sets acquired in systole and diastole. Brisk flow during systole results in the dephasing of arterial spins during the echo train and renders arteries dark. Conversely, arterial signal is preserved in diastole due to slow flow. Subtraction displays the arterial anatomy (Fig. 21.6). A commercially available technique for nonenhanced MRA using this labeling scheme is gated subtraction FSE imaging [9], which is also known as “Fresh Blood Imaging” (FBI), “Native Sampling Perfection with Application optimized Contrasts using different flip-angle Evolutions” (Native SPACE), “Delta Flow”, and “Trance”. The flow sensitivity of this technique can be tailored with partial Fourier sampling, adjustment of the refocusing flip angle, additional gradient lobes for flow compensation or spoiling, and by orientation of the frequency encoding axis. Specifically, reduced dephasing and signal loss is achieved with the use of partial Fourier acquisition, larger spin-echo refocusing RF pulse flip angles, flow compensation gradients, and by aligning the phase-encoding axis parallel to the arterial flow direction.
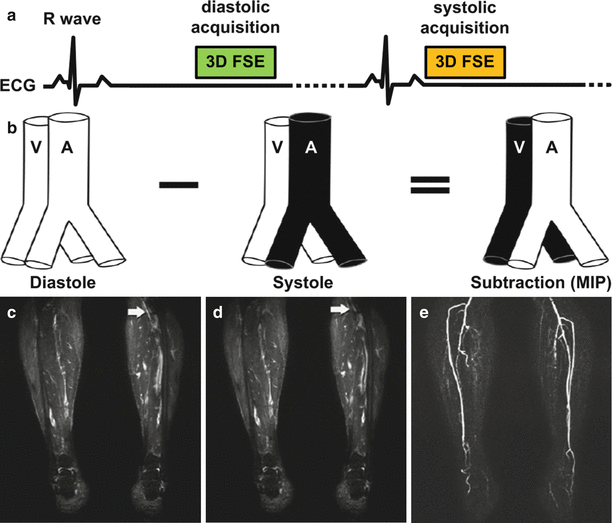
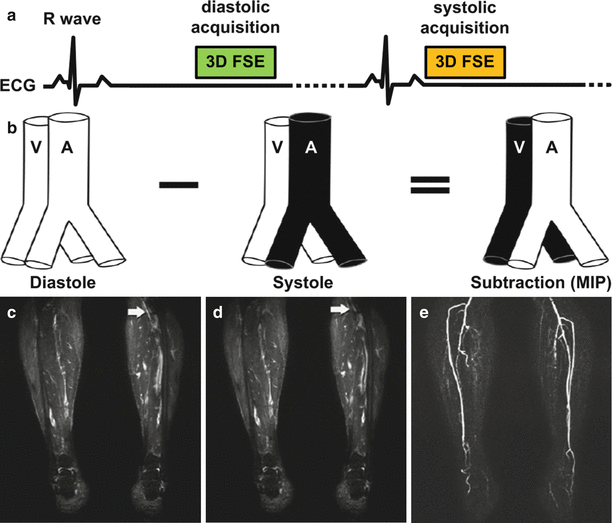
Fig. 21.6
Illustration of a cardiac-gated subtractive MRA sequence known as Native SPACE in the lower extremities. (a) The sequence consists of a 3D fast spin-echo (FSE) readout that is acquired in both diastole and systole. Typically, data from one cardiac phase (e.g. diastole) is acquired one slice-encoding step at a time until completion before acquiring data from the complementary cardiac phase (systole). The expected vascular signal of the sequence is shown in (b). Imaging gradients do not dephase arterial or venous signal in diastole when flow is slow (c), but dephase fast arterial flow in systole (d). Subtraction of systolic from diastolic images produces a nonenhanced MR angiogram (e) (Reprinted from Lim et al. [22] with permission from John Wiley and Sons)
With gated subtraction FSE imaging, dephasing of arterial spins during systole is accomplished by gradients within the imaging readout. Dephasing of arterial spins during systole may also be achieved by applying specially designed magnetization preparations before the imaging readout [10]. Figure 21.7 shows the design of one such sequence. Using RF and gradient pulses, these magnetization preparations spoil arterial signal based on its velocity or acceleration, leaving signal from stationary or non-pulsatile tissue unaltered. These approaches are most frequently applied before imaging readouts, such as bSSFP, which preserve arterial signal even during phases of substantial flow. Names of these techniques include “Flow Sensitive Dephasing” (FSD) and “Acceleration Dependent Vascular Anatomy for Non-Contrast-Enhanced MRA” (ADVANCE-MRA). With these methods, the degree of flow spoiling depends on the timing, duration, and strength of the gradient lobes applied. Gradients may also be applied along multiple axes to enhance flow spoiling and improve angiographic detail.
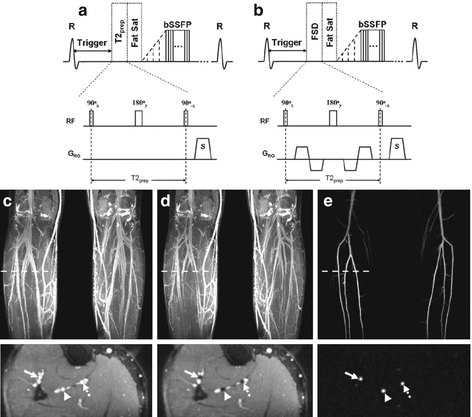
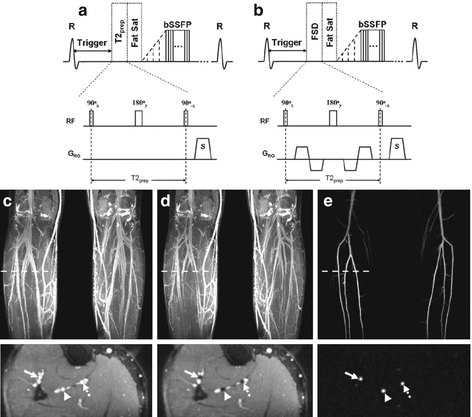
Fig. 21.7
Illustration of a gated subtractive 3D bSSFP MRA sequence known as flow sensitive dephasing (FSD) in the lower extremities. The sequence acquires data in (a) diastole and (b) systole; flow sensitization is applied by a FSD magnetization preparation that precedes image acquisition. When imaging is performed in diastole, the FSD preparation applies no dephasing gradients along the readout direction, which results in bright arteries and veins (c). Conversely, in systole, flow spoiling gradients are applied which results in arteries appearing dark (d); veins containing slow flow are not dephased and remain hyperintense. Subtraction of the imaging data produces a nonenhanced MR angiogram (e) with no background signal (Reprinted from Fan et al. [23] with permission from John Wiley and Sons)
Advantages, Limitations and Technical Considerations
Main drawbacks of ASL MRA include increased motion sensitivity and doubled scan time compared with non-subtractive nonenhanced MRA techniques. For scans requiring cardiac gating, knowledge of the systolic and diastolic phases of fast and slow flow is important to optimize image quality and avoid artifacts. This information can be obtained with phase contrast imaging or preparatory scans that survey several phases of the cardiac cycle. For gated subtractive techniques, abnormal flow patterns in patients with vascular disease can complicate the acquisition of dark-blood and bright-blood contrasts in systole and diastole, which can lead to image artifacts.
Some ASL methods for MRA require gating while others do not. In particular, ASL techniques based on differential RF pulses often do not require cardiac gating when coupled with readouts that preserve arterial signal during brisk flow, such as bSSFP. This is especially true in vascular beds containing predominantly continuous flow such as the carotid and intracranial arteries. Conversely, techniques that use imaging readouts that are sensitive to fast flow, such as FSE, generally require cardiac gating.
Applications of Arterial Spin Labeled Angiography
Although not often used in clinical practice, arterial spin labeling can be used to image a variety of vascular beds. In the evaluation of peripheral vascular disease without the use of contrast agents, gated subtractive FSE and flow dephased methods may be used. Of these methods, gated subtractive FSE imaging is the only technique that is commercially available. However, both gated techniques have good reported accuracy for imaging peripheral vascular disease in the distal lower extremities. Both gated techniques have potential for imaging the hands and feet, where contrast-enhanced MRA can be challenging due to slow arterial flow and short arterial to venous transit times.
In the evaluation of intracranial vascular disease, ASL can provide for time-resolved display of arterial flow patterns which may be helpful for assessing the functional significance of various disorders, including arterial occlusions, stenoses, and arteriovenous malformations. This information can be used to supplement the static MR angiograms obtained with 3D TOF MRA. In the carotid arteries, ASL can display arterial stenosis, but more validation is needed to assess the accuracy of the method and the merits and weaknesses of the technique in comparison with 2D and 3D TOF MRA.
Arterial spin-labeled MRA of the renal arteries is feasible but, as with the use of ASL in other vascular territories, large validation studies are lacking. In imaging the renal arteries, there is stiff competition from non-subtractive techniques such as inflow inversion recovery (IFIR) which are faster, less sensitive to motion, and have been better validated. ASL imaging has also been found capable of displaying portal venous anatomy.
Advanced Flow-Dependent Angiography
Overview and Physical Principles
Other than TOF and ASL techniques, a few non-subtractive flow-dependent nonenhanced MRA techniques have found increased utilization and development in recent years. Although these techniques still depend on the presence of flow for angiography, as is the case with traditional TOF MR angiography, they differ substantially from TOF in their implementation, timing and display of arterial anatomy. These methods are reviewed here.
Inflow Inversion-Recovery Angiography
Inflow inversion-recovery (IFIR) refers to a technique in which angiographic contrast is obtained by inflow of unsaturated arterial spins into the target vasculature and suppression of background spins through application of an inversion-recovery prepulse [11]. Figure 21.8 shows a timing diagram for such a sequence. Unlike traditional TOF MRA which applies a train of series of largely interrupted RF pulses separated by a short time interval, the IFIR method applies a slab-selective inversion pulse followed by a substantial inversion time (TI). This TI time, which is selected to ensure adequate inflow into the vascular anatomy under interrogation and an adequate degree of background suppression, is followed by a rapid imaging readout with a short interecho spacing.
< div class='tao-gold-member'>
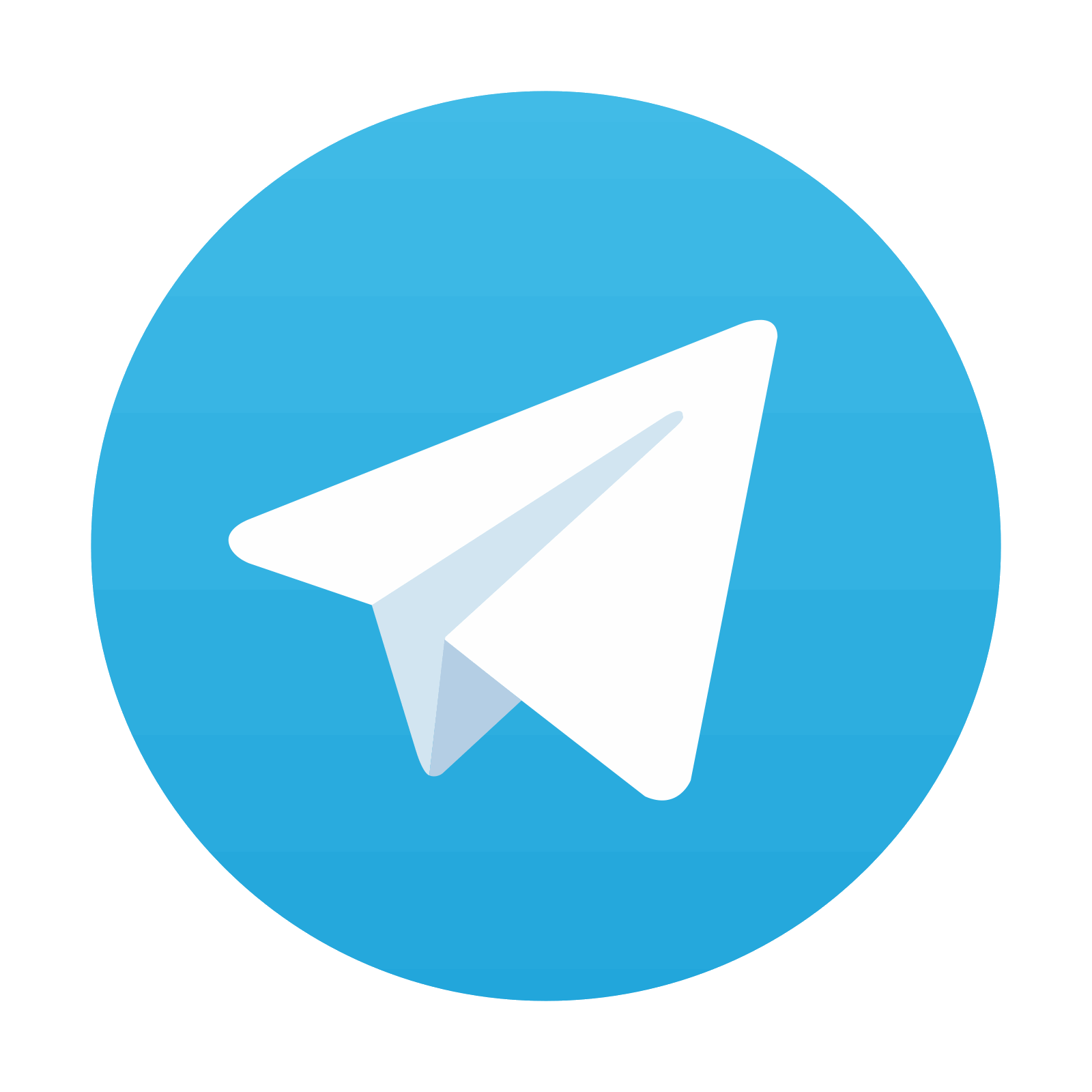
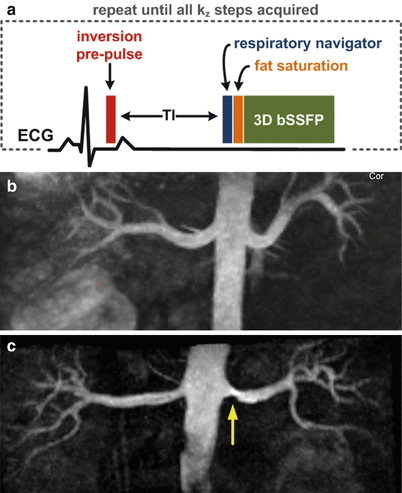
Only gold members can continue reading. Log In or Register a > to continue
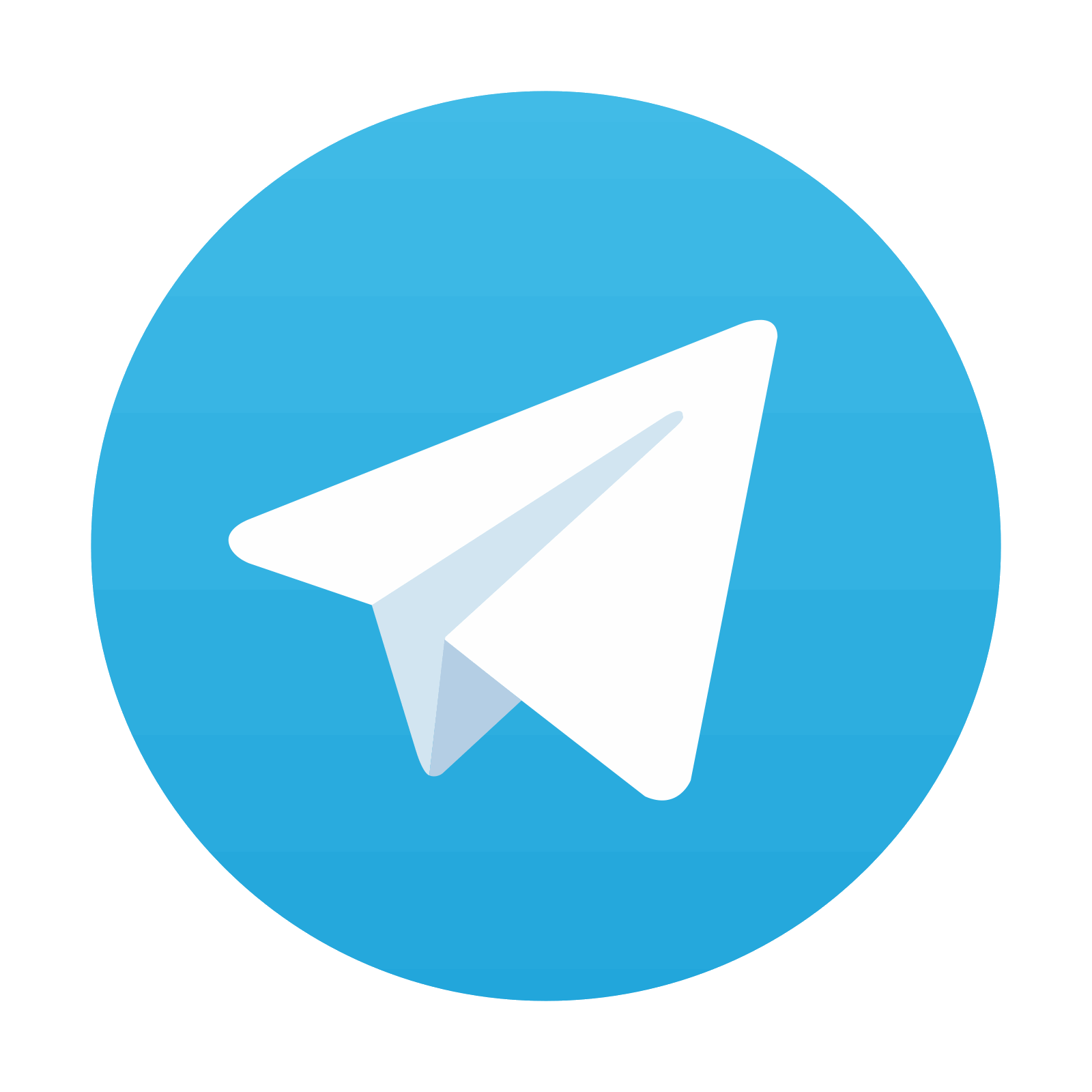
Stay updated, free articles. Join our Telegram channel
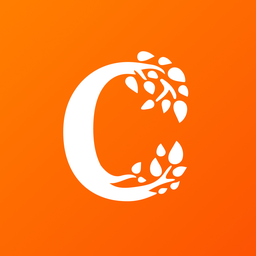
Full access? Get Clinical Tree
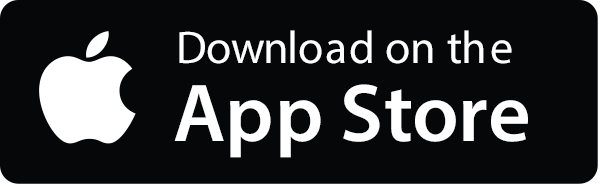
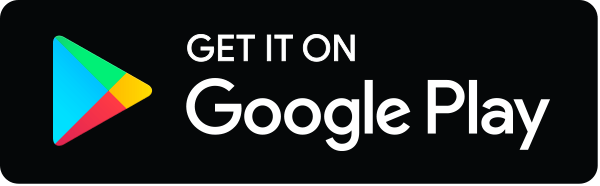