Introduction
Atherosclerosis is a lipoprotein-driven chronic inflammatory disease of arteries that leads to the accumulation of fibrotic, necrotic, and calcified tissue in the arterial intima, which is described as an atherosclerotic lesion or plaque. These plaques cause clinical disease by luminal narrowing or sudden precipitation of arterial thrombi that obstruct blood flow to the heart (coronary heart disease [CHD]), brain (ischemic stroke), or legs (peripheral artery disease [PAD]). The most common of these manifestations is CHD, such as stable angina pectoris and the acute coronary syndromes (ACS), including acute myocardial infarction (MI) (see Chapter 2 ).
Figure 3-1 shows a case example of ACS in a previously healthy 58-year-old man who suddenly developed chest pain and soon thereafter a lethal arrhythmia. The autopsy revealed an acute thrombus precipitated by an atherosclerotic lesion that had otherwise developed quietly, probably for many decades preceding the event. Each such fatal case is a missed opportunity. If the protagonist had known that the disease would lead to fatal complications, there would have been ways of retarding its development by lifestyle adjustments and preventive drug therapy (see Chapter 34 ). The key mechanisms underlying the development of atherosclerosis are currently known, and most clinical events should, in principle, be preventable if this knowledge is translated into effective preventive measures.

This chapter offers an introduction to the causes and central disease mechanisms of atherosclerosis, and describes what we know and have yet to learn about why some plaques suddenly precipitate life-threatening thrombosis. Furthermore, we discuss the terms plaque burden, activity, and vulnerability, which are commonly used to characterize the state and expected fate of individual lesions or patients. The pathobiology of myocardial injury, healing, and remodeling are discussed in Chapter 4 and Chapter 36 .
Multifactorial Causes
An increased blood concentration of apolipoprotein B (apo B)–containing lipoproteins, of which low-density lipoproteins (LDLs) is usually the most prevalent form, is necessary for atherosclerosis to develop, but many other factors can facilitate the development of atherosclerosis and MI (see Chapter 2 ). None of these other risk factors alone are sufficient to cause atherosclerosis, but because most individuals in modern societies have LDL levels that are permissive for development of atherosclerosis, the presence of other risk factors explains much of the occurrence of the disease. The central disease mechanisms discussed in the following sections are assumed to be the same, irrespective of the set of causal factors in a particular patient, but the presence of individual risk factors influences the course of the disease and the mode of presentation. For example, cigarette smoking predisposes to thrombotic complications and increases the risk more for MI than for stable angina, hypertension is an exceptionally powerful risk factor for stroke, and smoking and diabetes account for most of the risk of developing lower extremity PAD.
Mechanisms of Plaque Formation
The mechanisms leading to atherosclerotic plaque development are complex, involving lipoprotein retention, inflammatory cell recruitment, foam cell formation, apoptosis and necrosis, smooth muscle cell (SMC) proliferation and matrix synthesis, calcification, angiogenesis, arterial remodeling, fibrous cap rupture, thrombosis, and more ( ). Some of these are necessary steps in lesion progression (e.g., lipoprotein retention and vascular inflammation) and represent already exploited or potential targets for medical therapies. Other processes may be innocent bystanders (e.g., plaque calcification); although these are useful as a characteristic feature to identify atherosclerosis by imaging, they do not appear to be centrally involved in the genesis of lesions or their clinical complications. The combination of fat deposition and necrosis that lead to soft necrotic cores ( atheré : gruel or porridge [Greek]) and that of calcification and fibrosis that lead to hard tissue components ( scleros : hard [Greek]), distinguish atherosclerosis from other arteriosclerotic diseases, such as media sclerosis and arteriolosclerosis, and gave the disease its name.
It is important to realize that major parts of our understanding of the initiation and progression of atherosclerosis are deduced from studies in animal models. Experiments are necessary to demonstrate causal mechanisms, and although some experiments can be performed in humans or substituted by Mendelian randomization studies of randomly segregating gene variants, most are referred to exploration in animal models. Animals do not spontaneously develop atherosclerosis, but the disease can be induced in most species by increasing the level of LDL or other apo B–containing lipoproteins by feeding atherogenic diets or by genetic modification. Mouse models in particular have been essential because of the efficiency by which gene function can be probed in the living organism through genetic modification. Today our understanding of atherosclerotic lesion development in the mouse clearly surpasses similar insight into any other organism.
Knowledge of the disease in animal models is an important steppingstone for insight into human atherosclerosis, because the overall architecture of the disease processes is likely to be the same. However, it would be bold to assume that the molecular mechanisms are identical, and perhaps even more unlikely to think that the rate-limiting processes, and thus, the best targets for drug treatment, are conserved. Furthermore, because current models only feature some aspects of atherosclerosis, our mechanistic knowledge has important blind spots. There is in-depth knowledge of how LDL causes atherosclerotic lesion formation, but considerably less is known about the paths by which such lesions cause clinical disease through thrombosis or luminal narrowing, simply because this progression does not occur reproducibly in animals. Moreover, mechanistic insight into the effects of other causal factors, such as hypertension and diabetes, remains rudimentary.
Lesion Classification
Atherosclerosis is a progressive disease that begins early in life, but the speed of progression is highly dependent on vascular localization and varies markedly among different individuals. Even under the most facilitating conditions, it usually takes several decades to develop symptomatic lesions. The abdominal aorta, coronary arteries, ilio-femoral arteries, and carotid bifurcations are typically the most heavily affected.
By examining the same vascular sites in decedents of different age groups, pathologists have inferred a sequence of lesion development and have suggested criteria to classify lesions into types based on morphological criteria. Two classification schemes are commonly used. The American Heart Association (AHA) classification (types I to VIII) is based on a detailed microscopic analysis of human atherosclerosis at different stages of development and lends itself particularly well to studies that focus on the initiation and progression of lesions. A modified version, which is more direct in describing the link between lesion morphology and clinical complications, was later introduced by Virmani and colleagues ( Figure 3-2 ). A single patient with advanced disease will harbor many of these different lesion types across the vascular bed, reflecting the variability in the time of initiation, speed, and course of lesion development at different sites of the vasculature.

Lipoprotein Retention
LDL causes atherosclerosis by accumulating in the arterial intima. This accumulation does not occur uniformly throughout the vasculature, but is initially restricted to predilection sites near branch points and along inner curvatures. In these regions, the flow of blood exerts low or oscillatory shear stress on the endothelium, and these are further characterized by changes in endothelial turnover and gene expression, presence of subendothelial dendritic cells, and in humans, by the development of adaptive intimal thickening in the first months of life (see Figure 3-2A ). Intimal thickenings may grow to be as thick as the underlying media, consisting of a subendothelial proteoglycan-rich layer and a deeper musculo-elastic layer with SMCs and elastic fibers. It is tempting to speculate that these specialized areas of the vasculature serve physiological roles in vascular homeostasis or host defense in the normal body, but in the presence of supraphysiological levels of circulating LDL, they become the hotbed for atherogenesis. They are affected early during atherogenesis, and the rate of progression is higher here than at other arterial sites. With time, the disease spreads to the adjacent intima, and in older adult patients dying from MI, the epicardial coronary arteries are often “diffusively” affected by confluent plaques.
The ability of adaptive intimal thickenings to bind and retain insudating LDL particles from the blood may at least partly explain the propensity of these sites for the development of atherosclerosis. Studies in mice have revealed that local binding of LDL particles to proteoglycans is an important step in disease initiation, and extracellular lipid droplets in the proteoglycan-rich layer of adaptive intimal thickenings is the first microscopic sign of lesion development in children and young adults. As the disease evolves, the endothelium becomes more leaky, and the expression of bridging molecules (e.g., lipoprotein lipase) and proteoglycans with longer side chains promote the ability of the lesion to sequester LDL from the blood. This construct predicts that a higher LDL level is needed to induce the disease than to maintain and progress it once lesions have formed, and interestingly, is consistent with the strong relationship between LDL levels in young adulthood and the risk of developing CHD later in life.
Inflammation
LDL retained in the arterial intima is subject to oxidation and other types of modification, and thereby, acquires molecular epitopes (danger-associated molecular patterns) that are identical to or mimic epitopes on microbes and cell debris. These molecular epitopes are recognized by membrane-bound and cytoplasmic pattern recognition receptors and natural antibodies of the innate immune system. Adaptive immunity also reacts to modified LDL and mounts a multifaceted immune response. The endless supply of LDL from the blood and the formation of modified LDL in the intima provide a persistent proinflammatory stimulus that leads to chronic nonresolving inflammation in the vascular wall. Initially, endothelial cells and SMCs are induced to express adhesion molecules, chemoattractants, and growth factors that interact with receptors on monocytes and stimulate their homing, migration, and differentiation into macrophages and dendritic cells.
Foam Cells
In the intima, the recruited cells take up modified LDL through scavenger receptors and possibly by other mechanisms as well. This process clears modified LDL from the extracellular space, but leads to foam cell formation, with massive accumulation of cholesterol and cholesteryl ester droplets in the cytoplasm. Local SMCs also accumulate intracellular fat droplets, possibly by similar mechanisms.
Foam cell formation may itself be accompanied by proinflammatory activation, mediated by intracellular cholesterol crystals activating the NLRP3 inflammasome, but recruited macrophages may also be activated in a proinflammatory M1 direction by the binding of modified LDL to toll-like receptors. The activated cells in turn secrete proinflammatory cytokines (e.g., interleukin [IL]1-β and tumor necrosis factor-α [TNF-α]), reactive oxygen species and enzymes that promote further retention and modification of LDL (e.g., myeloperoxidase), and many other mediators that have been shown to play a role in atherosclerosis (e.g., plasminogen activators, cathepsins, and matrix-metalloproteinases). Other macrophages in the intima are polarized in an anti-inflammatory M2 direction and secrete proteins and small molecules (e.g., transforming growth factor-β and proresolving lipids) that favor resolution of inflammation.
T-helper 1 cells reacting toward modified LDL and other autoantigens related to the atherosclerotic process appear in the human intima coincident with the first foam cells, and they secrete proinflammatory cytokines (e.g., interferon-γ) that accentuate vascular inflammation in mouse models. However, other immune cell types, such as regulatory T cells and possibly B cells, may ameliorate it. In human, but not murine, lesions, cytotoxic T cells are abundant, albeit of unknown functional consequence to plaque development.
The description of the inflammatory response to modified LDL has become increasingly more complex with the recognition of multiple macrophage polarization phenotypes and T-cell subtypes, some with proinflammatory and some with anti-inflammatory activities. Importantly, as well as soothing to anyone trying to keep pace with the field, drastic LDL lowering has the potential to quickly resolve the inflammation. In atherosclerotic mice and rabbits that are reversed to normal LDL levels, plaque macrophages quickly reduce in number.
Fatty Streaks
Accumulating macrophage foam cells, which are easily recognized using a microscope, are telltales of lipoprotein-driven inflammation that occurs in the vascular wall. Foam cells initially accrue in the luminal, proteoglycan-rich layer of the intima, and when several layers have formed, they are visible to the unaided eye on the intimal surface as yellow-colored xanthomas or fatty streaks (see Figure 3-2B ). Xanthomas are harmless, and they are fully reversible. They are present in some infants in the first 6 months of life, probably reflecting the risk factors of the mother, but their number declines in subsequent years. At adolescence, they reappear in atherosclerosis-prone regions of the coronary arteries and the aorta in most people.
Necrosis
Many xanthomas do not progress further, but some, especially among those developing in adaptive intimal thickenings, develop necrotic foci with accumulation of acellular, lipid-rich material. Such lesions are collectively known as progressive atherosclerotic lesions and are further subdivided into pathological intimal thickening and fibroatheromas depending on the extent of necrosis. In pathological intimal thickening, only smaller lipid pools are present in the musculo-elastic layer beneath the layers of foam cells without gross disruption of the normal structure of the intima (see Figure 3-2C ). Such changes are commonly seen at 20 to 30 years of age in atherosclerosis-prone regions of the coronary arteries. In some lesions, the isolated lipid pools grow into confluent necrotic cores (also known as lipid cores), and large areas of the original intima are destroyed, probably by invading macrophages. Morphologically, this process can be characterized as being in an early or late stage of necrosis, with the former showing some presence of the original intimal matrix with macrophage infiltration, whereas in the latter, only a matrix-devoid, acellular gruel of lipids (cholesteryl esters, free cholesterol, phospholipids, and triglycerides) and cell debris are seen. When one or more necrotic cores are present, the lesion is a fibroatheroma (see Figure 3-2D ).
Death of SMCs and infiltrating macrophages are believed to be the main mechanism responsible for lipid pools and necrotic cores. Apoptotic SMCs and macrophages become detectable co-incident with the occurrence of acellular regions in human lesions, and cell death, both apoptotic and other forms, can be seen at the margin of the necrotic core. Many factors able to induce apoptosis in vitro are present in plaques (e.g., endoplasmic reticulum stress and oxidized lipids from modified LDL), and it is reasonable to assume that several of these cooperate to cause apoptosis in vivo. The presence of free apoptotic remnants in the tissue (i.e., not associated with phagocytic cells) indicates that impaired removal of apoptotic remnants (efferocytosis) contribute to the growth of the necrotic core. Instead of being phagocytosed by neighboring cells, remnants are left to undergo secondary necrosis, and the lipid-rich and proinflammatory cargo is consequently deposited in tissue.
The chemical composition of the necrotic core indicates that other sources of lipid may be important co-contributors, including direct accumulation of cholesteryl esters from insudating LDL and free cholesterol–rich erythrocyte membranes that are derived from intraplaque hemorrhages.
Necrosis of lesions is a critical part of lesion development, because it predisposes to clinical events. In its absence, the development of atherosclerosis would be a much less dangerous disease. Why necrosis occurs in some, but not other lesions, is not well understood, and apparently, the causal factors are at least partly dissociated from those that cause the initial xanthoma. For example, men and women develop similar amounts of coronary xanthomas early in life, but adult men have more lesions with lipid pools and necrotic cores than women of similar age.
Plaque Angiogenesis and Intraplaque Hemorrhage
The center of many atherosclerotic lesions become hypoxic, and cells respond by expressing hypoxia-inducible factor-1α (HIF-1α) and vascular endothelial growth factor, thereby providing a stimulus for the recruitment of neovessels into the base of progressive atherosclerotic lesions. The new vessels originate mainly from the adventitial vasa vasorum, and they are fragile and leaky, without supporting mural cells, giving rise to local extravasation of plasma proteins and erythrocytes, as well as providing an entry path for immune cells. Intraplaque bleeding from neovessels is common in fibroatheromas and may expand the necrotic core and promote inflammation. Another common source of plaque hemorrhage is extravasation of blood through a ruptured fibrous cap.
Lysis of red blood cells leads to spilling of free hemoglobin and heme moieties into tissues. Both components are oxidative and proinflammatory, and efficient defense systems have evolved to neutralize them. Free hemoglobulin is bound by haptoglobin, and the complex is internalized through CD163 in macrophages, whereas heme is bound by hemopexin and scavenged through the LDL receptor–related protein 1 (LRP1) in a variety of cell types. Inside cells, toxic heme is degraded by heme oxygenase-1, which leads to release of bilirubin and deposition of iron in the form of ferritin.
Histology shows that defense systems are in place to counteract the effects of intraplaque hemorrhage. Macrophages at sites of hemorrhage are polarized to a hemoglobin-scavenging type characterized by expression of CD163 and heme oxygenase-1. These cells lack typical markers of proinflammatory M1 macrophages (TNF-α and inducible nitric oxide synthase) and express the mannose receptor typical of M2-like macrophage differentiation, suggesting that they may dampen the proinflammatory effects of the hemorrhage. Lesions, especially fibroatheromas, also often contain abundant cellular ferritin.
The finding of associations between haptoglobin variants and cardiovascular disease supports the importance of an efficient defense against free hemoglobin in the atherosclerotic plaque. The human haptoglobin gene has a common polymorphism, which consists of an intragenic duplication of two exons. In addition to Hp1.1, which resembles haptoglobin in other mammals, it gives rise to larger multimeric haptoglobin variants, Hp2.1 and Hp2.2, which differ in their ability to neutralize hemoglobin, especially when hemoglobin is glycosylated. Several studies, although not all, have indicated that the cardiovascular risk in general, and the inflammatory response to intraplaque hemorrhage in particular, is accentuated in patients with diabetes who express the Hp2.2 variant.
Fibrosis
The connective tissue of lesions is initially that of the normal arterial intima or adaptive intimal thickening, but gradually this loose fibrocellular tissue is replaced and expanded by collagen-rich fibrous tissue, which often grows to become the quantitatively dominant component of plaques. Pieces of tissue that lie between a necrotic core and the surface of the plaque are called fibrous caps (see Figure 3-2 ).
The collagen, elastin, and proteoglycans of the fibrous matrix are mainly produced by SMCs, and the secretory function of lesional SMCs is reflected by their ultrastructural phenotype, characterized by an abundant rough endoplasmic reticulum and Golgi complex, and only sparse myofilaments. This phenotype has been termed synthetic in contrast to the contractile phenotype of medial SMCs. Few synthetic SMCs are present in the normal intima, but they increase substantially in number during lesion development, probably both by local proliferation and by migration of medial SMCs that subsequently undergo phenotypic modulation to the synthetic phenotype. Notably, the number of SMCs in plaques at all stages may be grossly underestimated because many synthetic SMCs do not contain detectable levels of the contractile proteins routinely used to recognize SMCs in tissue sections (e.g., smooth muscle alpha-actin [SMαA]).
Classical studies of X chromosome inactivation patterns found plaque SMCs to be arranged in large clonal populations, but these studies could not decide whether the precursor of these clones were patches of vessel wall with a single embryological cell origin, from which multiple SMCs migrated into lesions, or whether the atherosclerosis involved massive clonal expansion of a few SMCs. However, genetic tagging studies in the mouse indicate the last explanation is correct; SMCs undergo massive expansion during atherosclerotic lesion development. Marked cell proliferation may explain why plaque SMCs have shortened telomeres and express multiple other markers of senescence, and why the fibrous tissue eventually loses most of the SMCs that produce it, leaving behind large areas of hypocellular fibrosis.
Many plaques at autopsy consist exclusively of fibrous and sometimes calcified tissue without extracellular lipid pools or a necrotic core. The genesis of these fibrocalcific plaques is not fully understood (see Figure 3-2E ). Some pathologists believe that the development of a necrotic core is the prerequisite of fibrosis, and sequential sectioning often reveals that a necrotic core is present in the upstream or downstream vicinity of the section with fibrocalcific plaque. Where this is not the case, an originally formed core may have calcified or disappeared because of local quiescence of the atherosclerotic process or through silent plaque rupture with extrusion of the core (see the section on Mechanisms of Plaque Rupture ).
Calcification
Calcification is a characteristic feature of progressive atherosclerotic lesions and increases steadily with age. Microscopic hydroxyapatite granules are initially seen, especially in the basal parts of lesions within lipid pools and at the rim of developing necrotic cores. Matrix vesicles, apoptotic cell bodies, and cell debris from dying SMCs and macrophages in these areas appear to act as nidi for the initial calcium precipitation in a process resembling dystrophic calcification of other soft tissues undergoing injury. The dense calcifications that develop later mostly have an underlying acellular fibrous matrix without any signs of a necrotic core, inflammation, or angiogenesis.
Active cell-mediated processes akin to bone formation have been suggested to be involved in plaque calcification, but the structure of the initial calcification granulae is different from that of bone. However, cell-mediated processes may be involved in the subsequent conversion of the microgranulae to larger lumps and plates of calcium deposits, and osseous metaplasia are occasionally seen in lesions that are heavily calcified, sometimes including bone marrow. Interestingly, some studies suggest that statins promote coronary artery calcification.
Arterial Remodeling
During atherosclerotic lesion formation, the local vessel segment may either expand and thereby preserve or even increase the lumen (expansive remodeling) or shrink to diminish it (constrictive remodeling). Expansive remodeling is the general rule and explains why so few, even large, plaques cause severe stenosis. It may partly be a homeostatic response of the nondiseased vessel wall at sites of eccentric plaque formation to maintain normal shear stress. However, increasing evidence suggests that it is predominantly a pathophysiologic process in which proteolytic enzymes secreted by plaque macrophages cause the underlying media to thin and yield. This hypothesis is supported by the observation that plaque growth is frequently followed by a paradoxical increase in lumen area, and the fact that the direction and extent of remodeling is associated with the composition of the local plaque. Expansive remodeling is more often seen with fibroatheromas, and the extent of enlargement is positively correlated to plaque inflammation, medial atrophy, and the size of the necrotic core. The mechanisms underlying constrictive remodeling are not well understood, but it mostly occurs with fibrocalcific plaques and may be related to scar-like contraction of SMCs during plaque healing (see the section on Healed Plaques and Incorporated Thrombi). Interestingly, expansive remodeling may be reduced in patients with diabetes. The association between remodeling and atherosclerosis is a two-way process. As plaques develop and the arterial wall remodels as a result, local flow patterns change, and in turn, may influence the progression of the disease and ultimately the fate of lesions.
Because of remodeling, angiography is not useful for diagnosing the presence of atherosclerotic plaque or measuring changes in atherosclerotic plaque size with medical intervention (see Chapter 10 ). Similarly, single histological sections examined at autopsy cannot be used to make inferences about stenosis severity. As depicted in Figure 3-3 , the use of the term stenosis or histological stenosis in the pathology literature has little to do with the obstruction to blood flow.
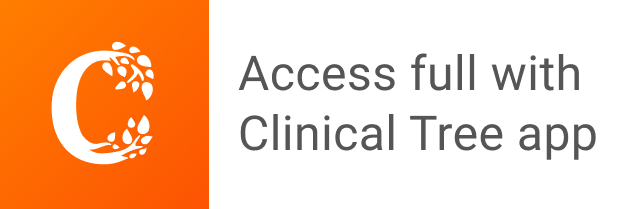