Figure 2-1.
Hierarchy for cardiac autonomic control. Autonomic control of cardiac function is mediated by a series of interacting feedback loops from the level of the heart [2] to that of the insular cortex [3, 4]. Cardiac afferents provide beat-to-beat sensory information about cardiac muscle activity to the cardiac autonomic nervous system [1]. The processing of these afferent neural signals at multiple levels of the hierarchy provides a mechanism for fine-tuned regulation of efferent neural signals in normal and stressed states [1]. DRG dorsal root ganglia, ICNS intrinsic cardiac nervous system (Adapted from Jänig [12]).
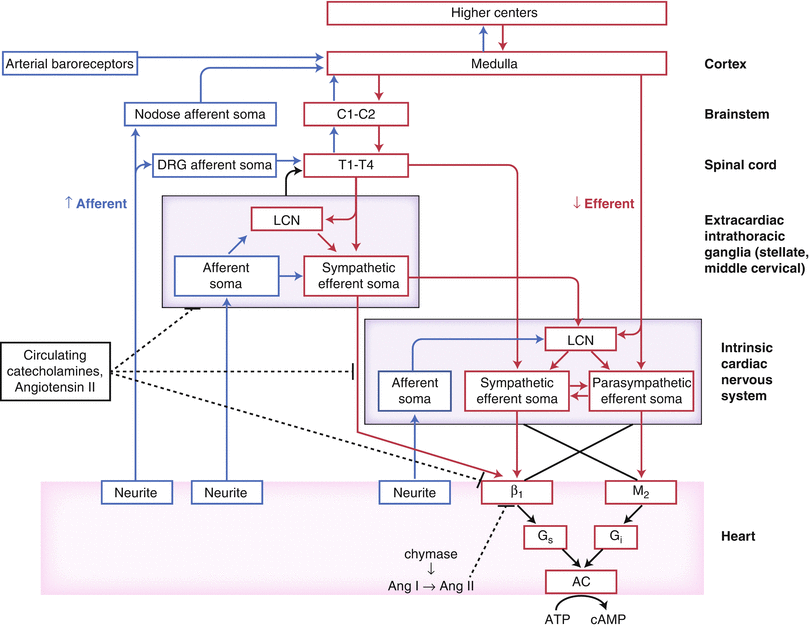
Figure 2-2.
Functional organization of cardiac innervation. This schematic shows neural network interactions between central and peripheral aspects of the cardiac autonomic nervous system. AC adenylate cyclase, Ang angiotensin, ATP adenosine triphosphate, C cervical, cAMP cyclic adenosine monophosphate, DRG dorsal root ganglion, LCN local circuit neuron, T thoracic (Adapted from Fukuda et al. [1]).
Cardiac Motor Neurons: Efferent Control of Cardiac Function
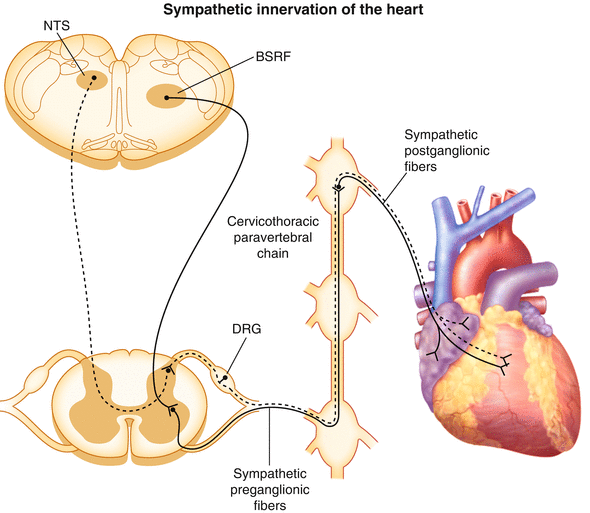
Figure 2-3.
Cardiac sympathetic innervation. The sympathetic nervous system is one of the two divisions of the autonomic nervous system that regulates cardiac function, the other being the parasympathetic nervous system. This diagram illustrates the path of sympathetic nerve fibers to the heart (solid line). Sympathetic preganglionic neurons innervating the heart originate in the intermediolateral cell column between the first and fourth thoracic segments of the spinal cord [13]. These preganglionic neurons receive inputs from glutamatergic neurons of the brainstem reticular formation (BSRF), which includes the rostral ventrolateral medulla [14]. Preganglionic neurons course through the ventral rami and synapse on postganglionic neurons contained primarily within extracardiac intrathoracic ganglia. Traditionally, it was thought that cell bodies of cardiac sympathetic postganglionic neurons were restricted to the stellate ganglia [15]; however, more recent data demonstrate that they also are found in the superior, middle cervical [16], mediastinal [17], and even intrinsic cardiac ganglia [2]. Postganglionic neurons from these ganglia innervate the atrial and ventricular myocardium, as well as the coronary vasculature. Preganglionic neurons release acetylcholine, which binds to nicotinic acetylcholine receptors on postganglionic neurons. Postganglionic neurons in turn release norepinephrine, which binds to α- and β-adrenergic receptors on cardiac myocytes and the coronary vasculature. This initiates a signal transduction cascade that involves G proteins, cyclic adenosine monophosphate, and protein kinase A, resulting in changes in chronotropy, dromotropy, inotropy, and lusitropy [13]. Also depicted in this schematic is one of the paths through which cardiac afferent signals are transmitted to the central nervous system (dashed line). These bipolar neurons with cell bodies in the dorsal root ganglia (DRG) have peripheral axons projecting to the heart and central axons projecting to the dorsal horn of the spinal cord. Second-order afferent neurons from the dorsal horn project to the nucleus tractus solitarius (NTS) of the medulla [18].
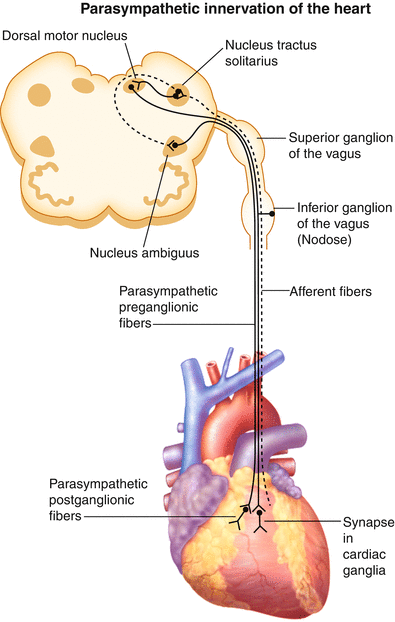
Figure 2-4.
Cardiac parasympathetic innervation. The parasympathetic nervous system is the other division of the autonomic nervous system that regulates cardiac function. This diagram illustrates the path of parasympathetic nerve fibers to the heart (solid line). Parasympathetic preganglionic neurons innervating the heart originate in the nucleus ambiguus and dorsal motor nucleus of the medulla oblongata in the brainstem [19]. In contrast to sympathetic preganglionic neurons, parasympathetic preganglionic neurons have long axons and synapse near the target organ. Cardiac parasympathetic preganglionic neurons synapse on postganglionic neurons contained within intrinsic cardiac ganglia [13]. Postganglionic neurons from these ganglia project axons to widespread regions of the heart [20]. Although most efferent nerve fibers contained within the vagus nerve are parasympathetic, sympathetic nerve fibers also have been reported [21, 22]. Preganglionic neurons release acetylcholine, which binds to nicotinic acetylcholine receptors on postganglionic neurons. Postganglionic neurons in turn release acetylcholine, which binds to muscarinic receptors on cardiac myocytes and the coronary vasculature [19]. The vagus nerve is also an important path for sensory information from visceral organs, including the heart, to the central nervous system. In fact, approximately 80 % of the fibers contained within the vagus nerve are afferent, being made up of A, B, and C fibers [23–25]. Cardiac-related bipolar neurons with cell bodies in the nodose ganglia have peripheral axons projecting to the heart and central axons projecting to the nucleus tractus solitarius of the medulla (dashed line) [19]. Thus, the vagus nerve contains both ascending afferent and descending efferent nerve fibers. Furthermore, as vagus nerve stimulation is evaluated as a potential therapy for a variety of chronic disorders, it is critical to understand the type of axons being activated in order to maximize the efficacy of the therapy while minimizing off-target effects (Adapted from Huang et al. [26]).
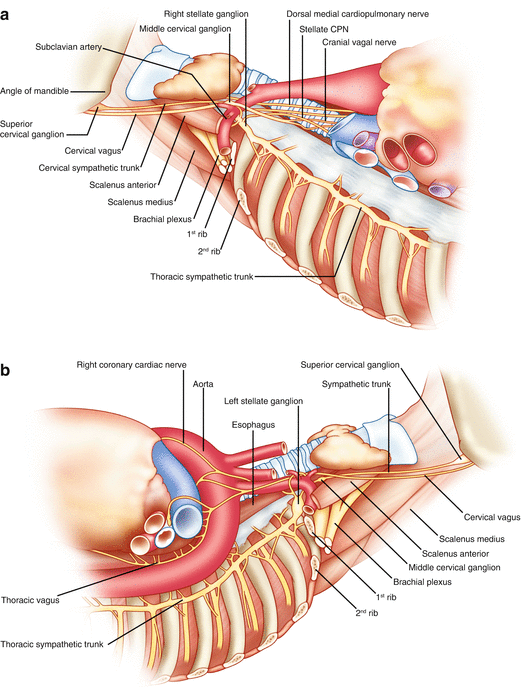
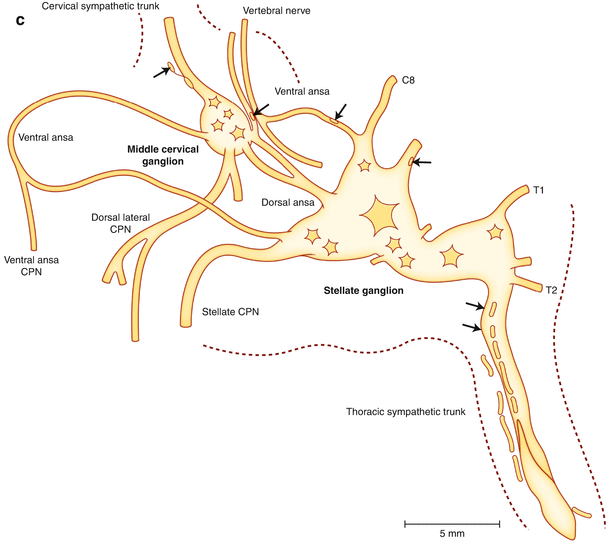
Figure 2-5.
Cardiac nerves from the neck and thorax. (a) Right aspect of the neck and upper thorax. This view shows the course of the right sympathetic trunk, the vagus nerve, and their major branches. As the sympathetic chain proceeds cranially, the first thoracic (T1) and inferior cervical ganglia (C8) fuse, forming the stellate ganglion. Two nerves from the stellate ganglion, the ventral and dorsal ansa subclavia, course around the subclavian artery as they travel to the middle cervical ganglion. The dorsal ansa may be absent on one or both sides because of merging of the stellate and inferior portion of the middle cervical ganglia [27]. The stellate and middle cervical ganglia contain most of the postganglionic sympathetic neurons that innervate the heart. Occasional ganglia called vertebral ganglia have been identified between the middle cervical and superior cervical ganglia, which are located at the base of the skull, near the angle of the mandible. In addition to the heart, sympathetic postganglionic nerve fibers contained in the extracardiac intrathoracic ganglia innervate the esophagus, trachea, and bronchi, as well as other structures in the head and neck [13]. There are multiple cardiopulmonary nerves (CPNs) that run lateral to the trachea as they course to the base of the heart. Sympathetic CPNs have been found to arise from the stellate ganglion and cervical sympathetic trunk below the level of the cricoid cartilage [27]. Parasympathetic CPNs arise from the right vagus nerve after the recurrent laryngeal nerve branches off at the subclavian artery. (b) Left aspect of the neck and upper thorax. This view shows the course of the left sympathetic trunk, the vagus nerve, and their major branches. Similar to the right side, the left stellate ganglion is located at the top of the thoracic sympathetic chain as a fusion of the T1 and inferior cervical ganglia (C8). The left vagus nerve courses on the anterior aspect of the aortic arch, and the recurrent laryngeal nerve branches off and travels under the aortic arch at the ligamentum arteriosum. Parasympathetic CPNs arise from the left vagus nerve at the pulmonary hilum.Figure 2-5. (c) Stellate and middle cervical ganglia. Axons from sympathetic postganglionic neurons contained in the stellate, as well as T2 through T4 ganglia, course through the ventral and dorsal ansa to the middle cervical ganglia as they travel to the heart. These sympathetic ganglia and nerves are covered in thick connective sheaths [27]. This is a composite drawing derived from several histologic sections of the stellate and middle cervical ganglia, along with the superior aspect of the thoracic sympathetic trunk, from human cadavers. The arrows indicate clusters of neuronal cell bodies, and dashed lines indicate the edges of connective tissue sheaths. (Adapted from Janes et al. [27]).
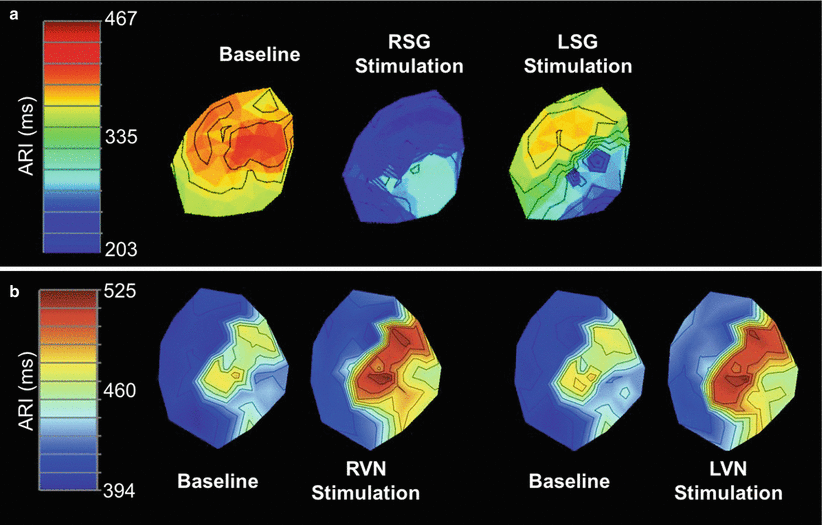
Figure 2-6.
Functional innervation of the heart. (a) Functional sympathetic innervation. Studies recording activation recovery intervals (ARIs), a surrogate measure of local action potential duration, have been used to determine functional innervation of the heart [28]. Data from such studies have demonstrated that neural projections arising from the right (RSG) and left stellate ganglia (LSG) innervate different regions of the heart. This figure is a polar representation of the ARI from the ventricular epicardial surface at baseline and during RSG and LSG stimulation. Stimulation of the RSG caused a greater shortening of ARIs on the anterior versus the lateral or posterior walls and on the base versus the apex, whereas stimulation of the LSG caused greater shortening on the lateral and posterior walls and the apex [29]. Stimulation of both the RSG and LSG caused an increase in dispersion of repolarization [30]. Thus, each stellate ganglion exerts regional influences. (b) Functional parasympathetic innervation. In contrast to the regional innervation by stellate ganglia, the right (RVN) and left vagus nerves (LVN) have homogenous effects on cardiac electrical and mechanical indices [31]. This figure is a polar representation of the ARI from the ventricular epicardial surface at baseline and during RVN and LVN stimulation. No significant differences in ARI were found among the anterior, lateral, and posterior regions of the ventricles [31]. In addition, stimulation of either the RVN or LVN resulted in similar changes in heart rate, left ventricular pressure, and left ventricular contractility [31]. One possible explanation for the homogenous pattern of innervation by the vagus nerves as opposed to the stellate ganglia may be its more prominent distribution through and interactions mediated within the intrinsic cardiac nervous system (Panel A adapted from Vaseghi et al. [29], panel B from Yamakawa et al. [31]).
Cardiac Sensory Neurons
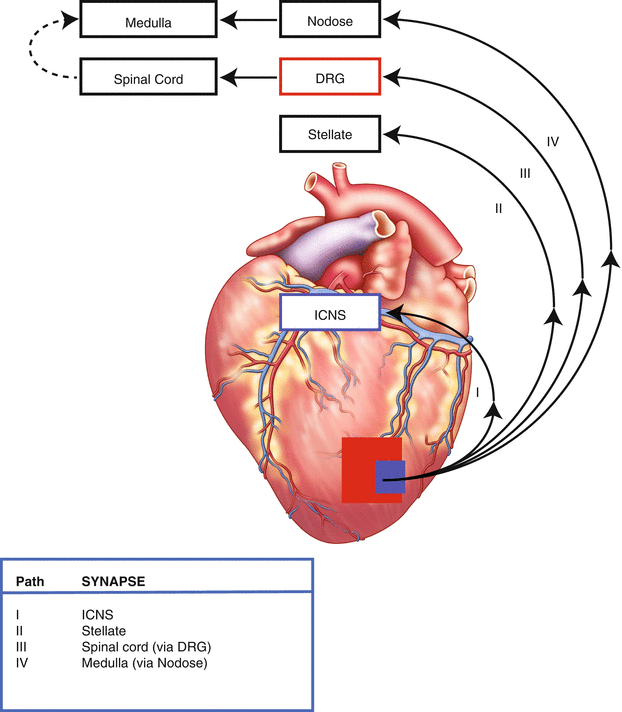
Figure 2-7.
University of California, Los Angeles (UCLA) classification of cardiac afferent pathways. This schematic shows the four paths through which sensory information from the heart travels to the central nervous system. Cardiac sensory (afferent) neurons are located at multiple levels of the cardiac autonomic nervous system, including intrinsic cardiac, stellate, middle cervical, mediastinal, nodose, and dorsal root ganglia (DRG) (Figs. 2.1 [12], 2.2 [1], 2.3, and 2.4 [26]) [18]. Bipolar neurons with cell bodies in the nodose ganglia have peripheral axons that project to the heart and central axons that synapse on second-order neurons contained in the nucleus tractus solitarius of the medulla [19]. Similarly, bipolar neurons with cell bodies in the DRG have peripheral axons that project to the heart and central axons that synapse on second-order neurons contained in the dorsal horn of the spinal cord [18]. Spinal cord neurons project to and interact with neurons in higher centers such as the medulla [32]. These afferent neurons transduce the local mechanical and chemical milieu of the myocardium and coronary vasculature [18]. Further, it has been shown that these neurons have multimodal transduction capabilities [2, 18]. A subpopulation of these neurons also transduce myocardial ischemia [18, 33–34], and thereby initiate a sympathoexcitatory reflex following myocardial infarction. ICNS intrinsic cardiac nervous system.
ICNS: Organ-Level Control
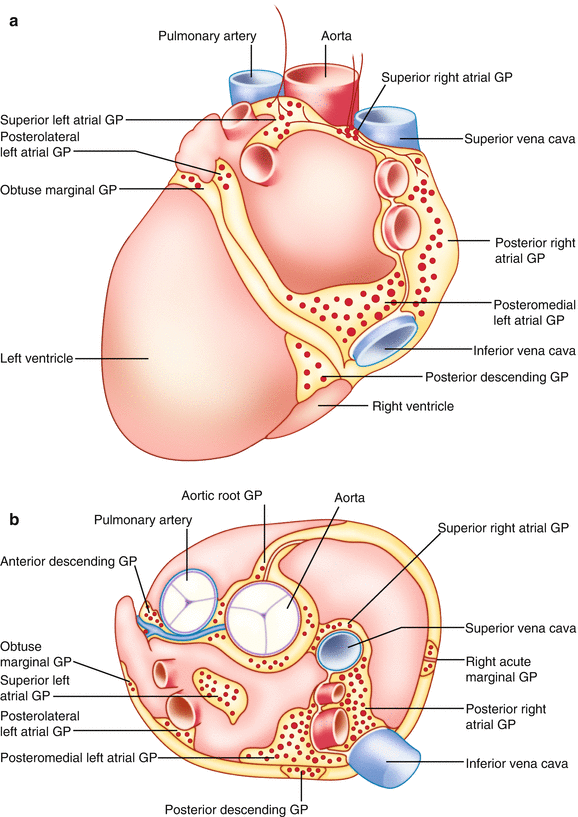
Figure 2-8.
Anatomy of the intrinsic cardiac nervous system. The autonomic nervous system projects to the heart via a distributed network of ganglia and interconnecting nerves located primarily in epicardial fat—the intrinsic cardiac nervous system [2]. Contrary to prior belief, the intrinsic cardiac nervous system is not simply a motor relay station for autonomic projections to the heart [35], but instead functions in concert with higher centers to modulate regional cardiac electrical and mechanical indices on a beat-to-beat basis [2]. The intrinsic cardiac nervous system is composed of a diverse population of neurons, namely sensory and motor neurons as well as interposed local circuit neurons [2]. Hence, the intrinsic cardiac nervous system has been referred to as the “little brain on the heart” [2]. An intrinsic cardiac nervous system has been identified in multiple mammalian species [36–38]. Intrinsic cardiac neurons are located in interconnected neuronal aggregates termed ganglionated plexi (GPs) (Fig. 2.9 [37]). The number and locations of GPs vary among species [36–38]. Anatomic studies of human hearts have identified major GPs in five atrial and five ventricular regions, with the atrial GPs located on the lateral and posterior aspects of the heart and the ventricular GPs located primarily at the base of the ventricles [37]. This schematic illustrates the location of the major GPs in the human heart on the posterior (a) and superior surface (b) of the atria and ventricles. Aside from these GPs, individual neurons and smaller ganglia have been found scattered through the atrial and ventricular myocardium [37] (Adapted from Armour et al. [37]).
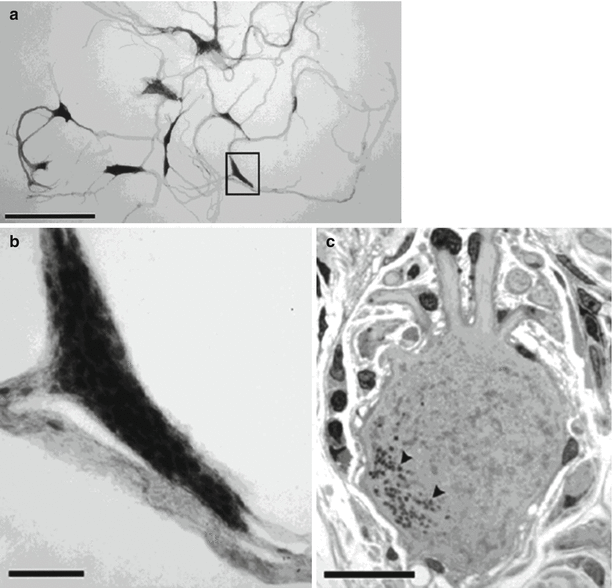
Figure 2-9.
Microscopic anatomy of the intrinsic cardiac nervous system. The human intrinsic cardiac nervous system is composed of approximately 14,000 neurons that are distributed through the ganglionated plexi [37]. Neurons contained within intrinsic cardiac ganglia share histologic similarities with those from other autonomic ganglia [37]. These figures are light photomicrographs from the posteromedial left atrial ganglionated plexus of a human heart, showing ganglia, nerves, and neuronal somata. (a) Network of ganglia and their interconnecting nerves stained with methylene blue. (b) Magnified view of inset from a illustrating a ganglion composed of approximately 150–200 neurons. (c) High-magnification view of a multipolar intrinsic cardiac neuron from the ganglion. Scale bars: 2.5 mm (a), 250 μm (b), and 25 μm (c) (Adapted from Armour et al. [37]).
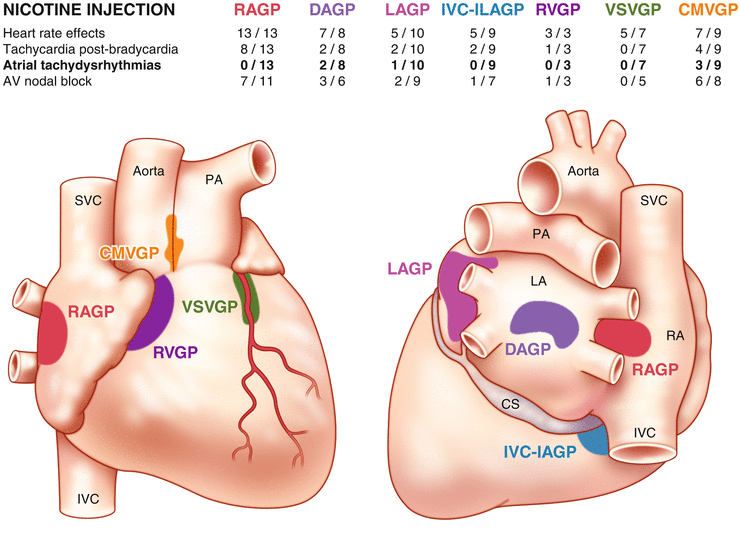
Figure 2-10.
Interconnectivity of the intrinsic cardiac nervous system. The intrinsic cardiac nervous system is composed of a diverse population of neurons, namely sensory neurons, motor neurons, and interposed local circuit neurons [2]. As previously described, these neurons are located within ganglionated plexi (GPs) that are found in distinct areas of the heart. Data for this figure were obtained by administering nicotine, which activates cholinergic receptors on intrinsic cardiac neurons, directly onto the epicardial fat in which a GP was located while recording evoked cardiac responses in a canine model [20]. This figure shows that each GP exerts preferential, but not exclusive, influence over specific regions. For instance, the right atrial GP (RAGP) primarily controls sinoatrial nodal function [39]; however, ventricular GPs, such as the ventral septal ventricular GP (VSVGP), still modulate heart rate [20]. This overlap in regions of influence is mediated by local circuit neurons. In addition, the presence of local circuit neurons allows for short-loop cardio-cardiac reflexes [2]. Local circuit neurons project axons not only to neurons contained within the same intrinsic cardiac ganglion but also to those in different intrinsic cardiac ganglia, extracardiac intrathoracic ganglia, and the central nervous system [2]. Local circuit neurons also receive diverse inputs [2]. The high degree of interconnectivity among these various neuronal populations implies the presence of local information processing [2]. The constant communication among these neurons also allows for dynamic modulation of cardiac output to meet the requirements of the body on both short and long time scales [2]. AV atrioventricular, CMVGP craniomedial ventricular GP, CS coronary sinus, DAGP dorsal atrial GP, IVC-ILA inferior vena cava-inferior left atrial GP, LA left atrium, LAGP left atrial GP, PA pulmonary artery, RA right atrium, RVGP right ventricular GP, SVC superior vena cava (Adapted from Armour [2]).
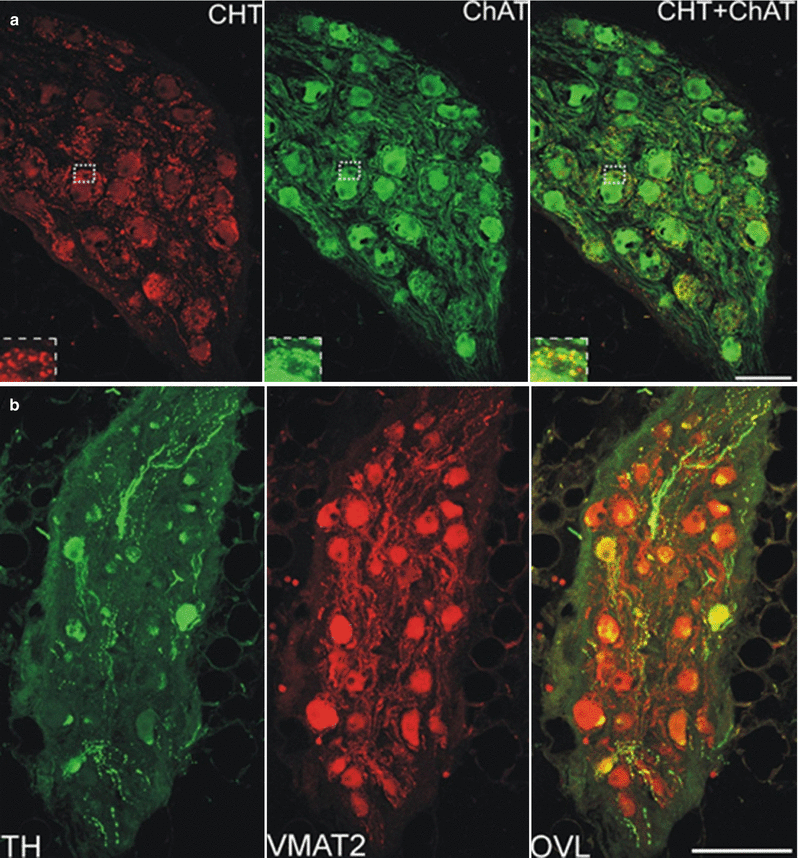
Figure 2-11.
Neurotransmitter profile of the intrinsic cardiac nervous system. A variety of neurotransmitters play a role in signaling within the intrinsic cardiac nervous system [40, 41]. Immunohistochemical studies have shown that neurons in human ganglionated plexi are positive for cholinergic, noradrenergic, and nitrergic markers, among many others [41]. This confocal microscopic image of an intrinsic cardiac ganglion from a human atrial ganglionated plexus shows the presence of neurons that display a cholinergic (a) and noradrenergic phenotype (b). The ganglion in (a) was double-labeled with choline transporter (CHT) and choline acetyltransferase (ChAT). CHT is required for high-affinity choline uptake by cholinergic neurons, and ChAT catalyzes the synthesis of acetylcholine. The ganglion in (b) was double-labeled with tyrosine hydroxylase (TH) and vesicular monoamine transporter 2 (VMAT2). TH catalyzes the rate-limiting step in catecholamine synthesis, and VMAT2 facilitates the transport of norepinephrine into storage vesicles. Interestingly, there are subpopulations of neurons that display colocalization of neuronal markers, further suggesting the ability of neurons within the intrinsic cardiac nervous system to transduce multimodal signals [41]. Furthermore, this provides strong evidence of the complex neurochemical anatomy that exists within this neural network, which goes beyond the classic view of the intrinsic cardiac nervous system as a motor relay station for parasympathetic preganglionic neurons. OVL overlay of TH and VMAT2 images. Scale bars: 100 μm (a) and 150 μm (b) (Adapted from Hoover et al. [41]).
Cardiac ANS in Disease
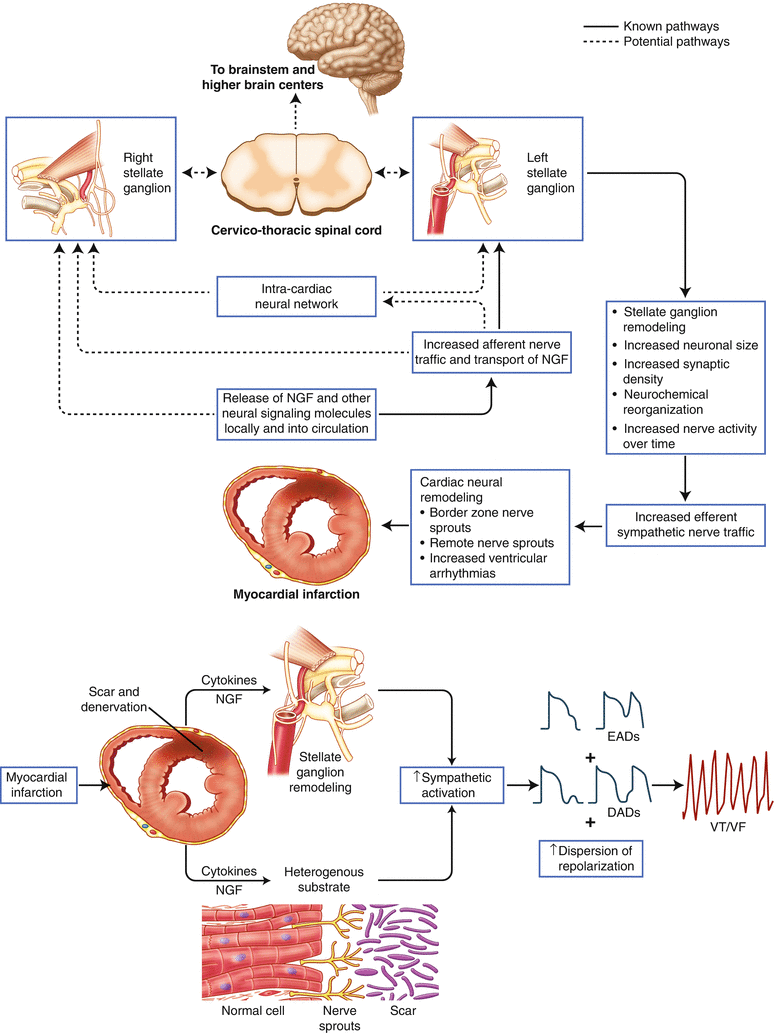
Figure 2-12.
Effects of myocardial infarction on the cardiac sympathetic nervous system. Cardiac disease, such as myocardial infarction (MI), has adverse effects not only on the myocardium but also on neurons at all levels of the cardiac autonomic nervous system [1]. Nerve injury following MI causes production and release of nerve growth factor (NGF) and other neural signaling molecules, leading to myocardial nerve sprouting [42]. Further, partial denervation of the infarct scar and hyperinnervation of the scar border zone create an arrhythmogenic substrate [43, 44]. Evidence also exists suggesting that there is retrograde axonal transport of these signaling molecules to extracardiac intrathoracic ganglia, such as the stellate [42]. There are both direct and indirect effects on neurons when the coronary artery blood supply is compromised, as is the case during MI. A lack of energy substrates and an accumulation of chemicals, such as adenosine and reactive oxygen species, secondary to the local ischemia cause altered activity of cardiac sensory afferent neurons [45–47]. Aberrant and excessive activation of these afferent neurons leads to reflex sympathoexcitation [48]. Acutely, this helps maintain cardiac output; however, chronically, it leads to adaptive and maladaptive remodeling, manifested as both structural and functional changes in neurons in both intrinsic and extrinsic cardiac ganglia (Figs. 2.13 [58], 2.14 [61], 2.15 [49], and 2.16 [62]) [1]. Porcine and human stellate ganglia neurons, for example, undergo morphologic and neurochemical changes following MI, such as an increase in neuronal size, synaptic density, and neuropeptide Y expression (Fig. 2.15 [49]) [50]. Adrenergic to cholinergic phenotype transdifferentiation has also been reported in rats with congestive heart failure [51]. Together with this structural remodeling, enhanced activity of stellate ganglia neurons has been shown to precede the onset of ventricular arrhythmias after MI in canine models [52, 53]. The extent to which the spinal cord and higher centers of the central nervous system remodel with cardiac injury and disease is not as well understood. However, studies using functional magnetic resonance imaging in humans have shown abnormalities in brain areas that mediate autonomic control in heart failure, such as the hypothalamus and insular cortex [54]. The heterogeneous myocardial and neural substrate created following MI is more prone to early afterdepolarizations (EADs) and delayed afterdepolarizations (DADs), as well as increased dispersion of repolarization, which may lead to ventricular arrhythmias [55, 56]. The heightened interplay between the sympathetic nervous system and the heart underscores the therapeutic value of acute and chronic use of β-blockers after MI (Fig. 2.17) [56]. For treatment of ventricular arrhythmias resistant to medical and catheter ablation therapies, the observed remodeling of the stellate ganglia lends support to the bilateral cervicothoracic sympathetic decentralization procedure (Fig. 2.18 [10, 57]) [11]. In contrast to the increase in sympathetic drive following MI, the mechanisms underlying parasympathetic withdrawal are less well understood. VF ventricular fibrillation, VT ventricular tachycardia (Adapted from Vaseghi et al. [56] and Ajijola and Shivkumar [55]).
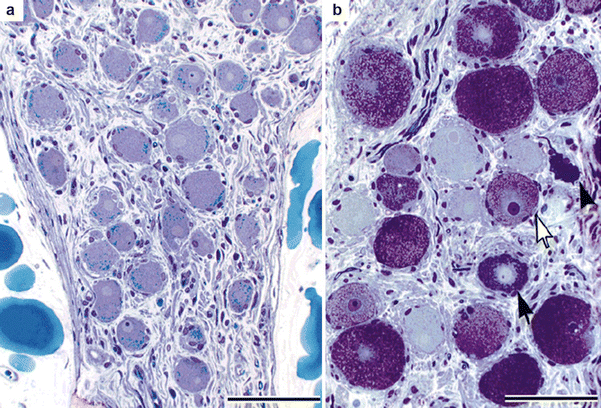
Figure 2-13.
Structural remodeling of intrinsic cardiac neurons in ischemic heart disease. Intrinsic cardiac neurons undergo histologic changes in patients with ischemic heart disease [58]. Neurons have been observed to contain lamellated inclusions and vacuoles, as well as to display degenerative changes in dendrites and axons [58]. Interestingly, the inclusions seen in these neurons are similar to those in central nervous system neurons of patients with metabolic diseases, such as lysosomal storage disease [59, 60]. This is a photomicrograph of intrinsic cardiac ganglia from the posterior right atrial ganglionated plexus of two patients with ischemic heart disease stained with toluidine blue. (a) Neurons in this ganglion have a normal appearance, with many lipofuscin granules and a pale, eccentrically located nucleus. (b) In another ganglion, many neurons are enlarged and filled with dark (black arrow) or lucent (white arrow) inclusions. One neuron (black arrowhead) that appears to be degenerating has very darkly stained cytoplasm and is misshapen. Scale bar: 100 μm (Adapted from Hopkins et al. [58]; with permission).
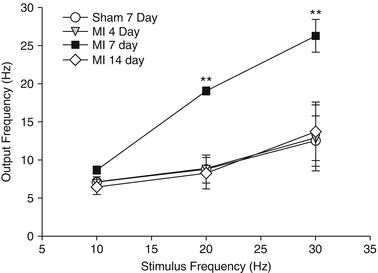
Figure 2-14.
Functional remodeling of intrinsic cardiac neurons after myocardial infarction. In parallel with structural changes, intrinsic cardiac neurons also undergo functional changes. An intracellular study of intrinsic cardiac neurons from a guinea pig chronic myocardial infarction (MI) model has shown that these neurons display alterations in basal action potential frequency, synaptic efficacy, and response to neuromodulators [61]. In this study, synaptic efficacy was assessed by stimulating axons (0.1–10 V, 2 ms, 10–30 Hz) synapsing on neurons with an extracellular electrode while concurrently recording their activity with an intracellular electrode [61]. This line graph displays the output frequency of the neurons at 4, 7, and 14 days post-MI and demonstrates that their synaptic efficacy is changed during the evolution of cardiac disease [61]. Points are mean ± SEM (n = 6 or more cells). This is most evident in the early stages following MI, as the output frequency was significantly greater in neurons from animals 7 days post-MI at 20 and 30 Hz compared with neurons from sham animals, as well as those from animals 4 and 14 days post-MI. Taken together, the adverse structural and functional remodeling of the intrinsic cardiac nervous system—the final common pathway for autonomic modulation of cardiac function—might be a mechanism underlying many cardiac pathologies and lead to a proarrhythmic state. **P < 0.001 by analysis of variance (Adapted from Hardwick et al. [61]).
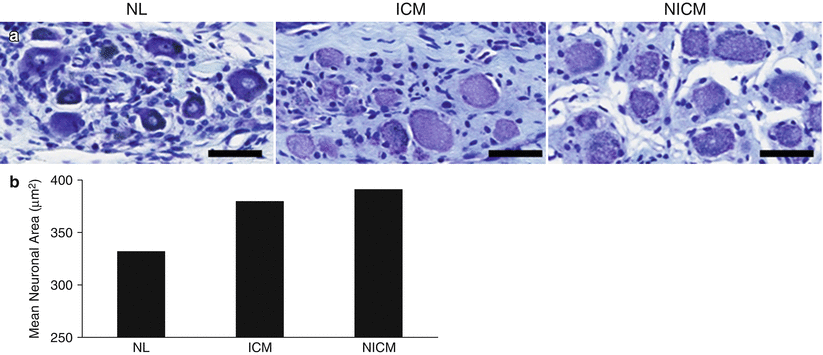
Figure 2-15.
Structural remodeling of stellate ganglia in chronic cardiomyopathy. Similar to intrinsic cardiac neurons, neurons from the stellate ganglia undergo morphologic and neurochemical changes in both animal models and humans with ischemic heart disease [49, 50]. This figure shows enlargement of stellate ganglia neurons from humans with ischemic (ICM) and nonischemic cardiomyopathy (NICM). (a) Stellate ganglia neurons stained with thionin from normal (NL), ICM, and NICM hearts. Scale bar: 50 μm. (b) Quantification of mean stellate ganglia neuronal size from normal, ICM, and NICM hearts. In addition to neuronal enlargement, synaptic density was significantly increased in stellate ganglia from NICM compared with normal hearts [49]. These findings may be a consequence of reflex sympathoexcitation resulting from myocardial injury (Adapted from Ajijola et al. [49]).
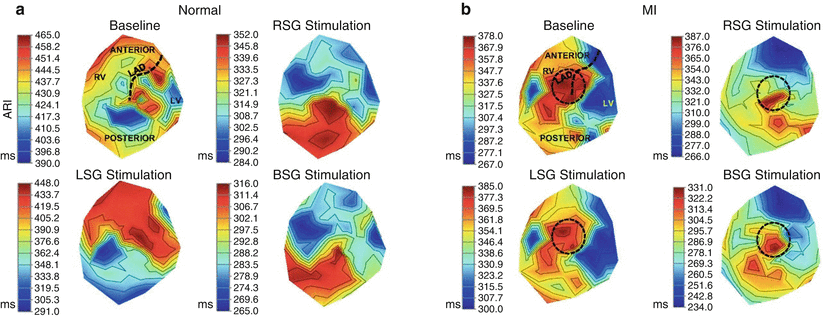
Figure 2-16.
Functional remodeling of sympathetic innervation after myocardial infarction. These structural changes manifest as alterations in functional sympathetic innervation of the heart, as determined by measuring activation recovery intervals (ARIs) in a porcine anteroapical myocardial infarction (MI) model [62]. In control hearts, right stellate ganglion (RSG) stimulation causes greater ARI shortening on the anterior surface and left stellate ganglion (LSG) stimulation on the lateral and posterior surfaces of the heart [30]. However, this pattern is lost following MI, in addition to an increase in dispersion of repolarization and alteration in activation propagation [62]. This figure is a polar representation of ventricular epicardial ARIs in control (a) and MI hearts (b) at baseline, as well as during RSG, LSG, and bilateral stellate ganglia (BSG) stimulation. Regions of the heart are delineated in the baseline condition of the control heart, and the location of the infarct is delineated by a black circle. These data indicate that a focal MI induces global remodeling of cardiac sympathetic innervation [62]. Furthermore, the heterogeneous pattern of ARI shortening seen post-MI may create a proarrhythmic substrate. LAD left anterior descending coronary artery, LV left ventricle, RV right ventricle (From Ajijola et al. [62]).
Cardiac ANS Neuromodulation
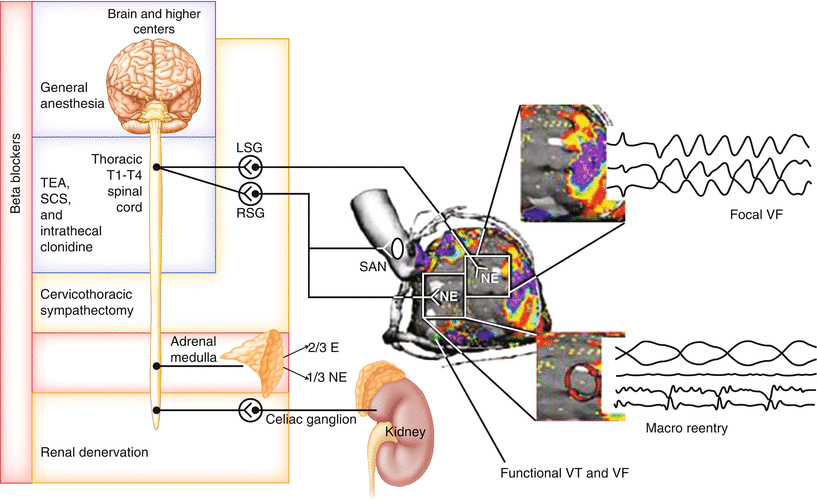
Figure 2-17.
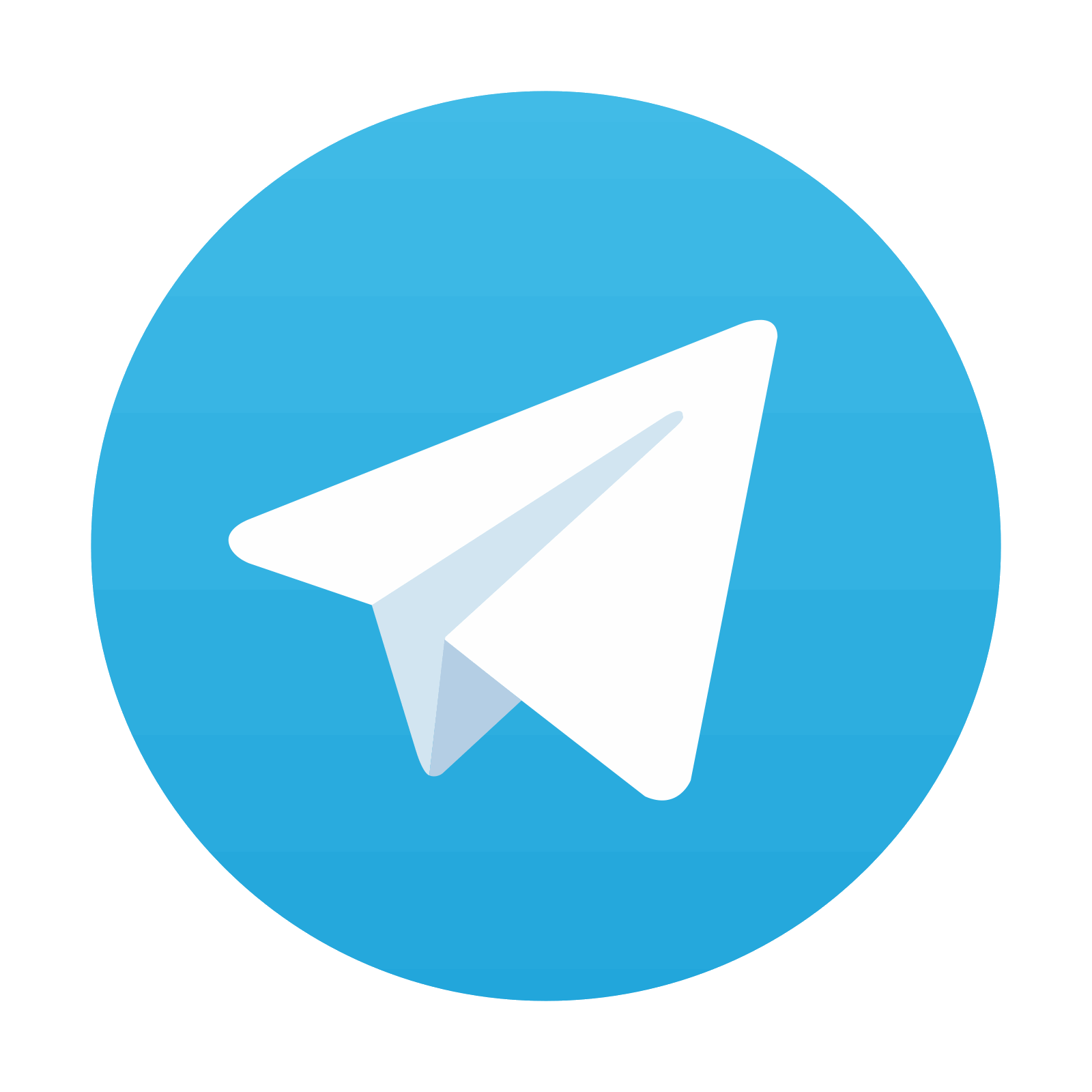
Targets of cardiac autonomic neuromodulation. This schematic depicts some aspects of the cardiac autonomic nervous system (ANS) that are being targeted via neuromodulation approaches for the treatment of cardiac diseases, especially arrhythmias and heart failure (HF). Intrinsic cardiac nervous system. Several mechanisms have been proposed for the pathogenesis of atrial arrhythmias, some of which involve the cardiac ANS [63]. A neural mechanism of atrial arrhythmias was first described in 1978 and has since been studied extensively [64]. Experimentally, stimulation of mediastinal nerves during the atrial refractory period has been shown to produce ectopic beats originating near the pulmonary veins and superior vena cava, which in turn may initiate atrial arrhythmias [65, 66]. It is now recognized that the cardiac ANS plays a major role in atrial arrhythmogenesis [67, 68]. The intrinsic cardiac nervous system has been proposed as a target in the treatment of atrial arrhythmias, and ganglionated plexus (GP) ablation is currently being used as an adjunctive or stand-alone treatment [69, 70]. Overall, however, GP ablation studies have yielded unclear results. The reasons for this likely are multifold. First, although each GP exerts preferential influence over specific regions of the heart, there is overlap among these regions (Fig. 2.10 [2]) [20]. This presumably ensures unperturbed neural control of cardiac function when one part of the network is compromised [2]. Second, most of these studies have relied on functionally identifying GPs to target energy delivery, on the basis of a bradycardic response to high-frequency electrical stimulation. The intrinsic cardiac nervous system is not composed solely of parasympathetic postganglionic neurons [2], and this method of identifying GPs fails to take into account the complex and heterogeneous composition of the intrinsic cardiac nervous system. Third, regeneration of neural structures may account for the return of atrial fibrillation incidence to baseline levels or worse in the long run [71]. Furthermore, there is evidence from a canine acute myocardial infarction (MI) model to suggest that ablation of atrial GPs may increase the vulnerability to ventricular arrhythmias [72]. Thus, a better understanding of the structural and functional complexity of the intrinsic cardiac nervous system is necessary before it is exploited as a therapeutic target. Vagus nerve. The vagus nerve is being targeted for disorders of the central nervous system (e.g., epilepsy, depression) [73], cardiovascular system (e.g., arrhythmias, HF) [8, 27], and gastrointestinal system (e.g., obesity) [74], among others. With regard to cardiac diseases, there is strong preclinical evidence supporting the use of vagus nerve stimulation (VNS) for the management of HF [8], and emerging data support its use for arrhythmias [27]. In a rat MI model of HF, VNS could attenuate adverse cardiac remodeling and improve long-term survival [75]. VNS-treated rats had reduced left ventricular remodeling, reflected by lower left ventricular end-diastolic pressures and higher left ventricular contractility [75]. The mortality rate also decreased from 50 to 14 % in the treatment group [75]. In another study in a canine pacing-induced HF model, VNS-treated dogs had improved ANS control of cardiac function, indicated by increased heart rate variability, baroreflex sensitivity, and reduced plasma norepinephrine levels [76]. Plasma angiotensin II and C-reactive protein levels also were decreased in the Figure 2-17. (continued) treatment group, suggesting that VNS may inhibit the renin–angiotensin–aldosterone system and exert an anti-inflammatory effect, respectively [76]. Based on the promising results of these preclinical studies, VNS has become the focus of three major HF clinical trials: CardioFit [77], Neural Cardiac Therapy for Heart Failure Study (NECTAR-HF) [78], and Autonomic Neural Regulation Therapy to Enhance Myocardial Function in Heart Failure (Anthem-HF) [9]. However, the results of these trials have been less clear, likely because of technical limitations in study design, including optimization of stimulation parameters. Aside from HF, VNS has a potential application in the management of arrhythmias, as it has been shown to stabilize cardiac electrical and mechanical indices [27]. In an acute canine model, VNS reversed the ventricular electrophysiologic changes induced by left stellate ganglion (LSG) stimulation, such as a decreased effective refractory period and action potential duration, as well as increased spatial dispersion of effective refractory period and maximum slope of the restitution curve [79]. Furthermore, in several animal models, VNS was shown to reduce the incidence of ventricular fibrillation and to increase the ventricular fibrillation threshold [27]. However, before this therapy moves forward clinically, a mechanistic understanding of the characteristics of the nerves being stimulated (afferent vs. efferent) and the potential impact of stimulation parameters (amplitude, frequency, pulse width, waveform, and duty cycle) is critical in order to ensure maximum efficacy while minimizing off-target effects [8]. Spinal cord. The spinal cord is a major convergence point for sympathetic and afferent inputs to and from the heart, respectively (Figs. 2.1 [12], 2.2 [1], 2.3, and 2.7). Thus, pharmacologic modulation (i.e., thoracic epidural anesthesia [TEA]) and electrical stimulation (i.e., spinal cord stimulation [SCS]) of the spinal cord represent another approach for the treatment of HF and arrhythmias [8, 57]. In a canine MI model of HF, SCS improved left ventricular function, reduced the incidence of ventricular arrhythmias, and slowed the progression of HF [80, 81]. Preemptive SCS reduced infarct size in a rabbit model of acute MI [82]. Similar results were found in a porcine model [83, 84]. These cardioprotective effects of SCS are thought to be the result of stabilization of reflexes at multiple levels of the cardiac ANS [8]. Kidneys. The activity of renal nerves may have significant effects on hemodynamic indices. Renal sympathetic nerves control renin secretion [85]. Renin causes activation of the renin–angiotensin–aldosterone system, leading to vasoconstriction as well as to sodium and water retention [86]. Aside from raising blood pressure, angiotensin II, the major hormone produced by renin–angiotensin–aldosterone system activation, has been shown to cause hypertrophy of cardiac myocytes [87]. Renal denervation is a potential treatment for arrhythmias and HF, in addition to drug-resistant hypertension [88, 89]. Because autonomic nerve fibers that innervate the kidneys run close to the renal artery [90], they can be targeted by surgical and catheter-based approaches. As previously described, MI causes denervation of the scar and hyperinnervation of the scar border and remote zones of the heart, as well as hypersensitivity to catecholamines (Figs. 2.12 [55, 56] and 2.16 [62]) [43, 44, 91]. The beneficial effects of renal denervation are thought to be through reducing afferent and autonomic efferent nerve activity. Although the results of preclinical studies and small case series of patients treated with renal denervation for ventricular arrhythmias have been encouraging, larger studies are needed [92–96]. Other novel approaches. Recently, novel approaches for HF management have emerged that leverage our increasing understanding of the cardiac ANS and its dysregulation in disease. For example, in a rat MI model of HF, epicardial application of resiniferatoxin, an ultrapotent agonist of transient receptor potential vanilloid 1, during MI induction attenuated adverse cardiac remodeling and improved cardiovascular dysfunction [97]. Because resiniferatoxin causes inactivation of sensory nerves, the mechanism in this study presumably is the prevention of afferent-mediated reflex sympathoexcitation. Overall, a better understanding of the cardiac ANS will allow development of rational neuromodulation therapies to manage cardiac disease. E epinephrine, NE norepinephrine, RSG right stellate ganglion, SAN sinoatrial node, T thoracic (Adapted from Vaseghi et al. [56]).
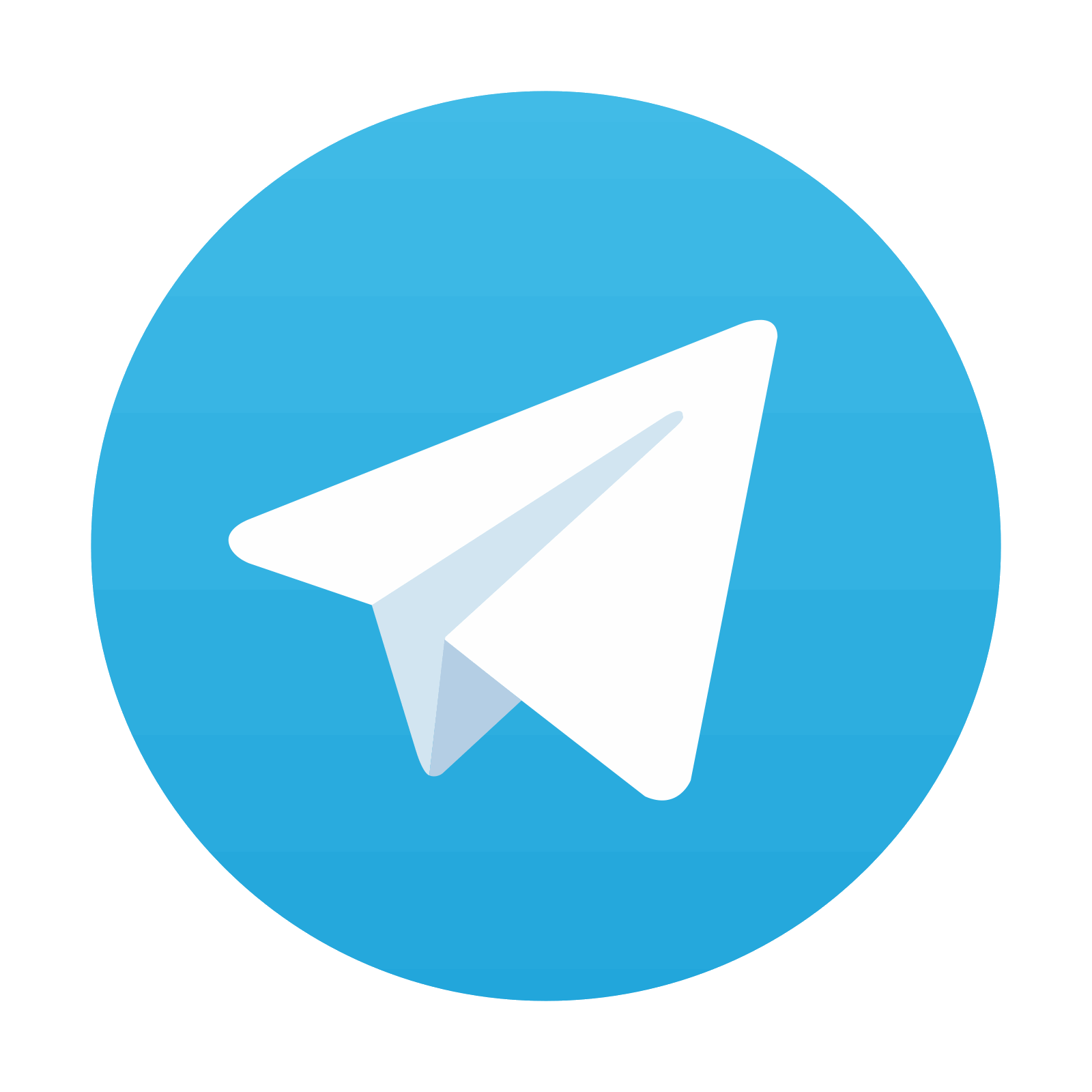
Stay updated, free articles. Join our Telegram channel
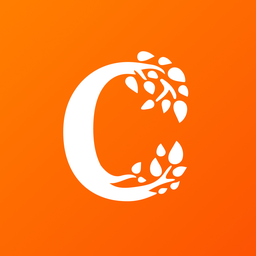
Full access? Get Clinical Tree
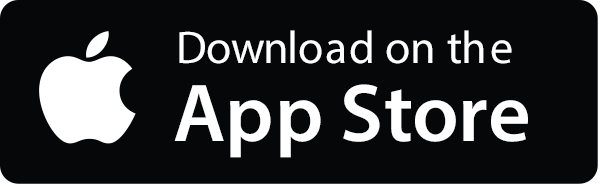
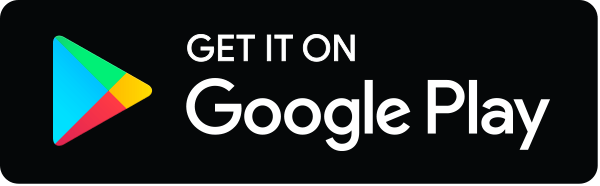
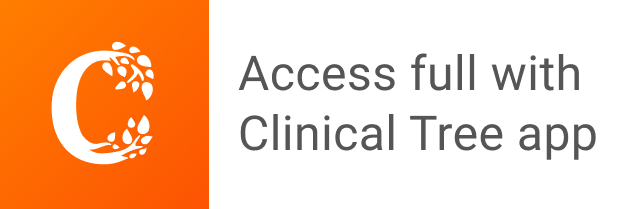