Myogenesis
Marc S. Penn
Amir Durrani
Ischemic heart disease remains the leading cause of congestive heart failure (CHF). The prevalence of CHF has increased dramatically over the preceding decade, with now more than 10% of the United States population over 65 years of age carrying the diagnosis. Because of the aging population, combined with the alarming increase in diabetes in the United States, the number of Americans with CHF is projected to increase to more than 6 million people by the year 2030.
Despite the improved outcome in patients with acute myocardial infarction (AMI) treated with mechanical reperfusion therapy, a substantial mortality and morbidity persists. Recent clinical trials of AMI that tested enhanced reperfusion strategies have not demonstrated significant improvements in mortality (1,2). Further complicating our attempts to minimize myocardial tissue loss in patients with AMI is the lack of significant progress in encouraging patients with symptoms of acute ischemia to present earlier. The time to presentation for patients enrolled in clinical trials is nearly 3 hours after symptom onset and has not changed over the past decade. These findings suggest that, for us to significantly improve the mortality of cardiovascular disease, a shift towards preserving and/or regenerating cardiac function following the ischemic insult must occur.
Because we may have reached a limit in our attempts to minimize myocardial necrosis using state-of-the-art reperfusion strategies in patients presenting with AMI, significant interest has evolved in optimizing or regenerating myocardial tissue following the ischemic event. Although the mechanisms are still under investigation (3, 4, 5, 6), the development of CHF following AMI involves more than just the loss of contractile tissue; CHF also is determined partly by the ventricular remodeling that occurs in response to myocardial necrosis. The inflammatory response to myocardial necrosis leads to infarct expansion, dilatation of the left ventricular (LV) cavity, and replacement of cardiomyocytes with fibrous tissue.
Currently available therapies to alter the remodeling process and the progression to CHF remain limited, and absolute death rates from CHF continue to rise. Furthermore, although cardiac transplantation is a definitive therapy for severe end-stage CHF, it remains limited in number and, in some instances, simply trades one set of symptoms for another. This unmet need of current therapies to preserve myocardial function following myocardial infarction (MI) has led to the identification of new treatment paradigms that focus on preventing myocardial cell death during MI, attenuating pathologic remodeling, and regenerating myocardium.
Autologous cell transplantation provides one mechanism through which this daunting task can be achieved (15). The goal of this review is to summarize the literature on cell therapy for the treatment of LV dysfunction, and to attempt to give a clinical perspective on the potential of this evolving therapeutic strategy.
Cell therapy is receiving growing attention as a potential therapeutic option to preserve and/or regenerate contractile tissue and counter ventricular remodeling. To combat heart failure using cell therapy, the objective is to reverse myocardial thinning, dilatation, and decreased function by replacing a critical number of lost cardiomyocytes with contractile cells transplanted into the infarct zone (7). Cellular transplantation has focused on several distinct cell types for use in experimental and first clinical pilot trials, including differentiated cells such as skeletal myoblasts, cardiac myocytes, smooth muscle cells, and cardiac fibroblasts. Other studies have focused on the viability of stem cells derived from the bone marrow, embryonic, and umbilical chord tissue (8, 9, 10, 11). Currently, the primary questions being addressed by experimentation are (a) Which cells (or combination of cells) are best suited for cardiac regeneration and vascularization?; and (b) Which signals can be used to recruit them? (10). Moreover, cell survival must be optimized once engraftment has taken place. The answers to these questions are complex and take into
account practical and safety concerns. Additionally, the different routes of cell delivery via intravenous infusion, or catheter-assisted intracoronary and intramyocardial injection, can produce varying outcomes (12, 13, 14). Concurrent clinical investigations will help to assess the impact that cell therapy has on either preserving or improving cardiac function during or following AMI.
account practical and safety concerns. Additionally, the different routes of cell delivery via intravenous infusion, or catheter-assisted intracoronary and intramyocardial injection, can produce varying outcomes (12, 13, 14). Concurrent clinical investigations will help to assess the impact that cell therapy has on either preserving or improving cardiac function during or following AMI.
STEM CELLS
Stem cells are self-renewing, undifferentiated cells that maintain an ability to differentiate into cells with different end-organ functions. A series of experiments recently established that adult stem cells may have the ability to differentiate into various cell lines such as cardiomyocytes, epithelial cells, hepatocytes, neurons, and pancreatic cells and refuted the long-standing notion that the number of these organ-specific cells is determined at birth and that the body is not capable of renewing them. Increasing evidence also suggests that many organs contain endogenous or local pools of stem cells that may express organ-specific markers (15,16). Whether these stem cells are continually or periodically renewed by the bone marrow-derived stem cells is still unknown. Different types of adult stem cells have been shown to differentiate into endothelium, smooth muscle cells, and cardiomyocytes, hinting at the possibility of myocardial regeneration (17); however, the origin of these cells is highly controversial (18).
Embryonic Stem Cells
Embryonic stem (ES) cells are continuously replicating cell lines derived from an embryonic origin isolated from the blastocyst inner cell mass (19,20). The properties of ES cells include derivation from the pre- or peri-implantation embryo, prolonged undifferentiated proliferation with conditional constraints, and the ability to form tissues derived from all three germ layers. When they are properly cultured, ES cells expand at a rapid rate and group to form embryocidal bodies that have the ability to differentiate into a wide variety of specialized cells, including cardiomyocytes (21). Three stages of differentiation are observed in the cultured cardiomyocytes: primary myocardial, intermediate, and terminal (atrial-, ventricular-, nodal-, His- and Purkinje-type cells). These cell types and stages are distinguished on the basis of electrophysiologic, histochemical, and morphologic properties; some of these cells exhibit enhanced selection based on culture conditions (22, 23, 24).
The use of ES cells for myocardial regeneration still requires a great deal of additional investigation. The transplantation of human ES cells into immune-deficient rats has the potential to induce the formation of teratomas; and intramyocardial transplantation of cardiac myocytes derived from ES cells exhibit arrhythmic potential (25). ES cell commitment to the cardiac lineage promotes analysis of developing heart cells. The in vitro differentiation of ES cells provides a powerful technique to investigate early cardiomyogenesis, differentiation of early cardiac precursor cells, the development of excitability, excitation-contraction coupling, and the molecular signals that occur in these processes. This line of research is ultimately critical for our understanding of not only ES cells, but also to optimize adult stem cell therapies. Autologous umbilical cord blood-derived stem cells could be sequestered and applied in the future for a number of uses. However, practical limitations include harvesting, possibility of rejection, and high risk of teratocarcinoma. Ethical constraints also continue to limit investigation.
Adult Autologous Cells
A variety of adult human tissues contains stem cells that have been shown to potentially have the ability to lead to recovery of cardiac function under the proper conditions, with or without the ability to differentiate into cardiomyocytes. The application of these autologous cells, which includes bone marrow-derived stem cells (hematopoietic and mesenchymal stem cells), skeletal myoblasts, and adipocytes, for therapy is appealing because they are easily harvested, do not illicit an immune response, and do not rouse ethical concerns.
Skeletal Myoblasts
Skeletal myoblasts (SKMB) are skeletal muscle mononuclear satellite cells, normally found in a dormant state beneath the basal membrane of the skeletal muscle fibers. After tissue injury, these cells promote repair and regeneration by rapidly recruiting, proliferating, and fusing at the site of injury. Skeletal myoblasts form mature myofibers as they repopulate an area of fibrosis, but they do not transdifferentiate into cardiac myocytes (18,26). Although the engrafted SKMB express slow β-myosin heavy chain and acquire other characteristics of slow twitch muscle, the expression of the major proteins involved in mechanical and electrical integration—N-cadherin and connexin-43, respectively—are down-regulated postimplantation (27). It is not certain whether engrafted SKMB contract in unison in vivo (26), although they are able to contract via exogenous stimulation. As such, improvement of overall pump function could rely on other mechanisms, such as stretch-induced contraction of transplanted SKMB resulting from the action of cardiomyocytes or the field effects of these cardiomyocytes (direct transmembrane channeling of electrical currents) (28). The primary mechanism by which SKMB exert beneficial effects may lie in their ability to generate a scaffold that releases growth factors to inhibit matrix-producing pathways and stimulate the matrixdegrading pathways that encourage antifibrotic effects, thereby attenuating remodeling (29,30).
SKMB are the most studied cells to date in clinical trials for the treatment of ischemic cardiomyopathy. Early data
suggest that in territories to which SKMB were introduced, increased wall thickening occurs. Unfortunately, high doses (on the order of 800 million cells or greater) have been shown to be arrhythmogenic, with four out of the ten patients experiencing ventricular tachycardia requiring implantable defibrillators (31). Whether this arrhythmogenesis was due to SKMB awaits the completion of placebo-controlled randomized trials.
suggest that in territories to which SKMB were introduced, increased wall thickening occurs. Unfortunately, high doses (on the order of 800 million cells or greater) have been shown to be arrhythmogenic, with four out of the ten patients experiencing ventricular tachycardia requiring implantable defibrillators (31). Whether this arrhythmogenesis was due to SKMB awaits the completion of placebo-controlled randomized trials.
Studies have demonstrated that SKMB transplantation can serve as an effective strategy for gene transfer. Most commonly, SKMB engineered to secrete angiogenic proteins have been shown to significantly increase angiogenesis (32,33) and decrease infarct size if delivered at the time of MI. Furthermore, we recently have demonstrated in a model of ischemic cardiomyopathy that the delivery of adenovirus-encoding vascular endothelial growth factor (VEGF) is significantly more efficacious using SKMB as vehicles of delivery than via direct viral injection (34). Ultimately, a significant limitation of SKMB therapy is that obtaining sufficient cell numbers to impart a clinical effect is a time- and labor-intensive process. Therefore, it is unlikely that SKMB-based therapy will ever develop into a therapy that can be delivered at the time of myocardial infarction.
BONE MARROW-DERIVED STEM CELLS
Hematopoietic Stem Cells, Progenitor Cells
Hematopoietic stem cells (HSC) comprise 1% to 2% of the adult bone marrow. HSC transplantation is able to permanently reconstitute the entire hematopoietic system. HSC maintain the ability to differentiate into lineages of blood-forming cells, some of which are able to differentiate into cells contained in myocardial tissue (e.g., endothelial cells) (11,17). In addition to the CD34+ HSC that are used to reconstitute hematopoietic cells to treat hematopoietic ailments, CD45+, CD117+ (or c-kit+), and CD14+ markers also characterize subpopulations of HSC. Recent studies suggest that the CD34+ and CD117+ subpopulation demonstrates bone marrow (BM) stem cell plasticity (35,36). The assumption is that the plasticity of this population permits environmental signals generated by the myocardium to encourage transdifferentiation of these cells into cardiac myocytes or endothelial cells postengraftment (11,17). Unfortunately, emerging data indicates that these cell types do not differentiate into cardiac myocytes.
Mesenchymal Stem Cells and Multipotent Adult Progenitor Cells
Stromal progenitor cells comprise less than 0.05% of the adult bone marrow. Mesenchymal stem cells (MSC) and multipotent adult progenitor cells (MAPC) form subpopulations of bone marrow stromal progenitor cells; both populations are able to differentiate along multiple lineages (39, 40, 41). MSC can be immunoselected via CD45 negativity and cultured through as many as 40 population doublings before attaining senescence (42). Makino and Fakuda have shown that 5-azacytidine, a DNA demethylating agent, can induce MSC to transdifferentiate into cardiac myocytes in vitro (43). Rat and swine infarction models indicate that MSC may have the ability to differentiate into cardiac myocytes according to site specificity, when precultured with 5-azacytidine (44). Moreover, MSC differentiation into blood vessel cells coordinated with MSC differentiation into cardiac myocytes result in improved regional perfusion and wall motion, increased scar thickness, and enhanced global heart function. Shake et al. implemented a proprietary preculturing technique and avoided using 5-azacytidine, because it raises clinically relevant safety concerns. After directly injecting autologous MSC into the infarct zone of swine hearts, Shake et al. found that the MSC engrafted in the host myocardium and demonstrated expression of muscle-specific, but not cardiac-specific, proteins. In the same model of left ventricular wall infarction, MSC engraftment may have helped to attenuate contractile dysfunction and pathologic thinning (43).
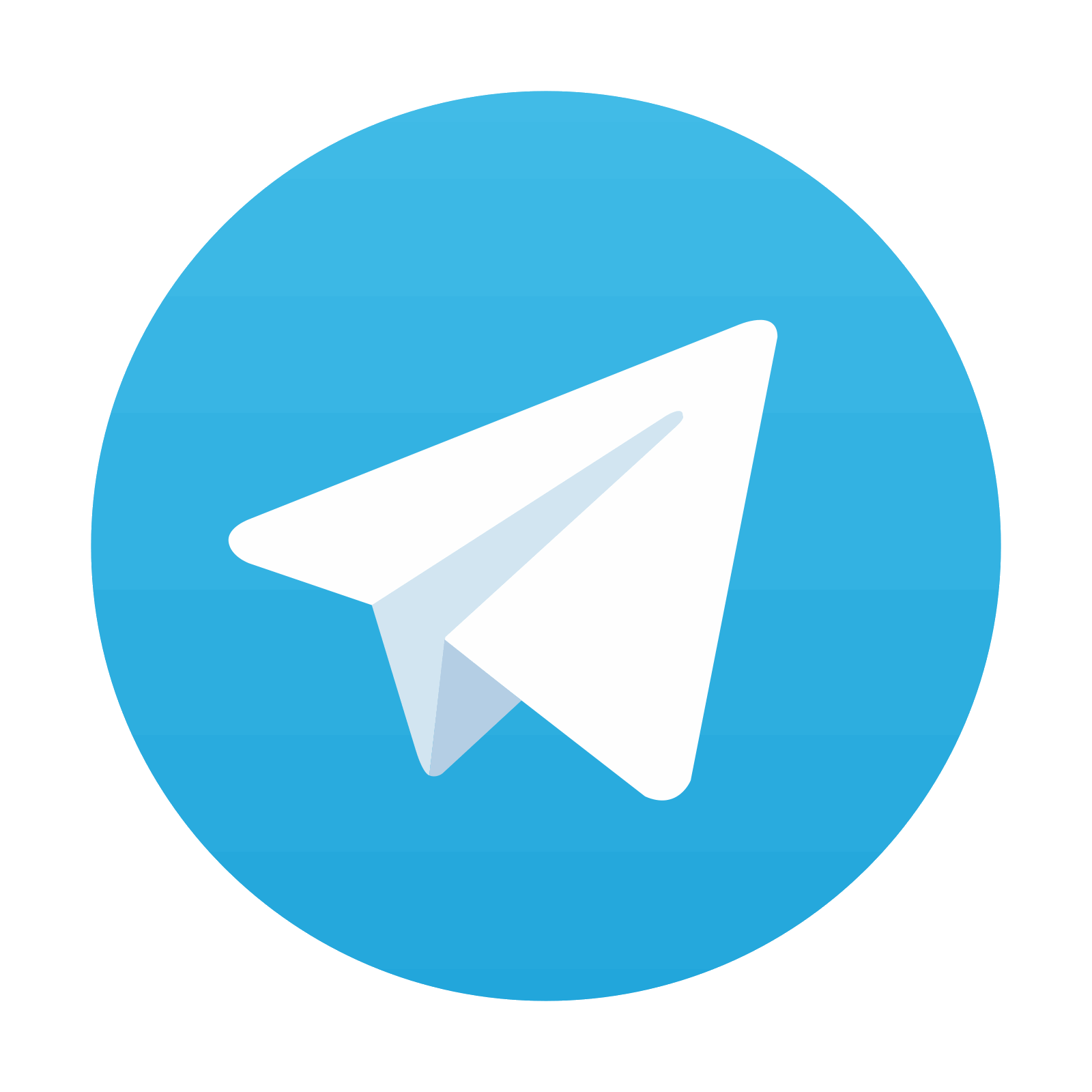
Stay updated, free articles. Join our Telegram channel
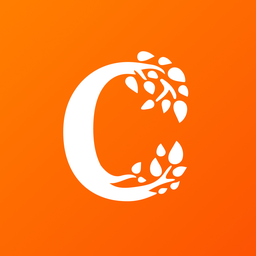
Full access? Get Clinical Tree
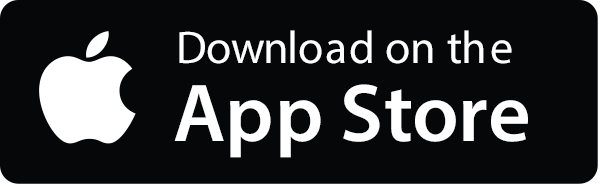
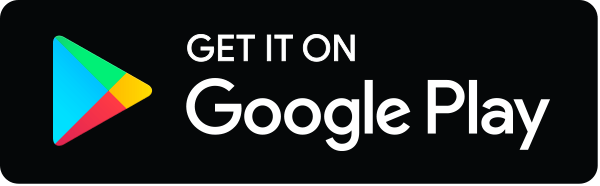