As described in Chapter 1, the developing mammalian heart undergoes a series of complex and tightly regulated processes during structural organogenesis. Considerable understanding of the genetic control of these pathways has been gained in recent years. Of equal importance are the functional changes in cardiac contraction and relaxation that accompany morphological development of the cardiovascular system. However, compared to our understanding of structural organogenesis, much less is known about the genetic, molecular, and cellular processes that control cardiac contractile function during embryonic development and fetal maturation.
Although age-related changes occur in the functional cardiac responses to pharmacological or physiological interventions, understanding of the underlying mechanisms is generally incomplete. In particular, there is relatively little known about fundamental mechanisms of excitation-contraction coupling and regulation of contractile function in the immature human heart. A thorough understanding of the basic molecular and cellular processes governing contractile function is essential for development of rational and age-appropriate pharmacological strategies for fetal and neonatal patients with impaired cardiac contractile function.
It should be noted that current concepts of molecular and cellular aspects of myocyte function during cardiac development and maturation are derived mainly from animal models. Limited information is available regarding these processes in the immature human heart. The list of suggested readings at the end of this chapter refers to several monographs that provide a comprehensive and detailed overview of contractile function in the mature heart. In addition, references are listed that provide additional information regarding developmental changes in cardiac ultrastructure, metabolism, electrophysiology, and responses to pathophysiological states. Integration of these myocellular changes into a larger perspective of developmental physiology and cardiac mechanics is presented in Chapter 3.
Excitation, contraction, and relaxation of myocardial cells are mediated by complex ion transport processes and coordination of calcium delivery to and from the contractile proteins (Figure 2-1). At rest, active transport processes (mainly the sodium-potassium pump [Na/K-ATPase]) maintain electrochemical gradients across the sarcolemmal membrane. Consequently, a resting membrane potential is established with the cell interior being negative relative to the extracellular space. Depolarization of the cardiac sarcolemmal membrane occurs largely due to opening of sodium channels, which results in a rapid influx of sodium and a brisk rise in membrane potential from negative to positive values. As described in more detail below, this change in membrane potential is ultimately translated into an increase in intracellular cytosolic calcium, binding of calcium to the contractile protein complex in the myofibrils, and cell shortening (contraction). Relaxation occurs as the resting sarcolemmal membrane potential is re-established, intracellular cytosolic calcium decreases, and calcium dissociates from the contractile protein complex.
FIGURE 2-1.
Schematic diagram of the major components involved in calcium transport, excitation-contraction coupling, contraction, and relaxation in mature mammalian ventricular myocytes. At rest, a negative membrane potential is maintained largely by the action of the sodium-potassium pumps. Contraction in adult myocytes (see also Figure 2-5) is triggered by membrane depolarization (opening of sodium channels), which then promotes opening of voltage-dependent L-type calcium channels. The resulting influx of a relatively small amount of calcium causes the release of a large amount of calcium from the junctional sarcoplasmic reticulum by triggering the opening of specific sarcoplasmic reticulum calcium release channels. This process is termed “calcium-induced calcium release.” The central role of T-tubules in providing the proper spatial orientation for close coupling of L-type calcium channel calcium influx to sarcoplasmic reticulum calcium release is depicted. The rise in cytosolic calcium results in calcium binding to troponin C, activation of the myofilaments, and contraction. The predominant mechanism for lowering calcium to promote relaxation (see also Figure 2-7) is the ATP-dependent reuptake of calcium into the longitudinal sarcoplasmic reticulum via the actions of sarcoplasmic reticulum calcium pumps, which are in turn regulated by the phosphorylation state of phospholamban. During steady state, the same amount of calcium that enters the cell is extruded, mainly by the sodium-calcium exchanger. The β-adrenergic receptor/G protein/adenylyl cyclase complex is illustrated to signify the central role of this system in regulating cardiac contraction and relaxation (see also Figure 2-8).

These processes must be very tightly regulated to maintain calcium homeostasis and control of contraction and relaxation. During the past several years, it has become clear that many of the pathways and proteins involved in these processes undergo developmental regulation. Consequently, significant age-related differences exist in the fundamental mechanisms of cardiac contraction, relaxation, and regulation of contractile function.
As noted above, most of our knowledge regarding developmental aspects of cardiac contractile function is derived from animal models, such as chickens, rats, rabbits, mice, and zebra fish. A recurring problem in developmental cardiology relates to extrapolating animal studies to human physiology. Because cardiac development and maturation are controlled by genetic and epigenetic factors, there may be species-specific events that are unique for a given animal model. It is therefore useful to compare results from more than one animal model. Studies using human myocardium would provide more relevant results, but the availability of tissue for these types of research is limited, especially for fetal and neonatal human hearts.
In the human embryo, the elements required for rhythmic contraction and relaxation of the heart become functional by approximately 3 weeks after conception. The major functional units of the developing cardiac myocytes are the sarcolemmal membrane, sarcoplasmic reticulum, mitochondria, and contractile proteins. At the same time, supporting structural components (fibroblasts and the extracellular matrix) are also developing. Each of these elements undergoes progressive development and maturation throughout embryonic, fetal, and early postnatal life. In the early embryo and fetus, the ultrastructural appearance and spatial arrangement of cellular structures are quite different compared to those of the fully mature heart.
Ventricular myocytes change considerably with regard to size, shape, and overall appearance during the transition from late fetus to the adult. In general, the perinatal maturation phase is characterized largely by the addition of cellular structures and more precise spatial organization of the elements involved in contraction and relaxation. Newborn myocytes exhibit random orientation of myofibrils with incomplete sarcomeres. Myofibrils are frequently located in the subsarcolemmal region. Regularly scattered throughout the cell are ribosomes, rough endoplasmic reticulum, and mitochondria. Nuclei are round and centrally located. Relative to cell volume, nuclear volume decreases steadily after birth, coincident with progressive cellular hypertrophy. The clusters of ribosomes, rough endoplasmic reticulum, and extensive Golgi apparatus are all consistent with active protein synthesis during rapid cell growth.
Although the general patterns are similar, the precise timing and temporal relationships of the ultrastructural changes vary from species to species. Table 2-1 summarizes comparisons among various species for the timing of appearance and maturation of important elements involved in cardiac contraction and relaxation. The information presented in Table 2-1 is somewhat generalized because maturation is not uniform throughout the same heart within a given species. Furthermore, considerable variation in morphologic appearance of myocytes can be found within the same heart.
Species | Appearance of T-Tubules | Maturation of Sarcoplasmic Reticulum |
---|---|---|
Rat | 21 days of age | 1 to 11 days of age |
Rabbit | 8 to 10 days of age | 5 to 14 days of age |
Hamster | 16 days of age | 1 to 30 days of age |
Guinea pig | Complete at birth | Complete at birth |
Dog | 60 days of age | Postnatally |
Human | 32 weeks’ gestation | First appearance at 30 weeks’ gestation; functional maturation unknown |
An important change during postnatal development is a progressive decrease in surface area–to–volume ratio. This is due largely to a progressive increase in myocyte volume during postnatal development. Myocyte division (hyperplasia) is commonly observed during fetal growth and in the early newborn period. However, shortly after birth, continued growth of the heart is attributable largely to hypertrophy of existing myocytes, with relatively slow turnover and cell division. The mechanisms involved in this postnatal switch from hyperplastic to hypertrophic growth are incompletely defined at present. A number of laboratories are working to unravel the basis for this fundamental change in myocyte biology since the implications for cardiac repair and regeneration are profound.
In cardiac myocytes, the sarcolemmal membrane is well defined throughout fetal and postnatal development, and the glycocalyx can be visualized quite early in cardiac development. Transverse tubules (T-tubules) are invaginations of the sarcolemma into the cell that allow the extracellular environment to extend into the inner cellular structures. In species with small ventricular myocytes (eg, birds and fish), the T-tubular system is generally not present, presumably because it is not required for efficient excitation-contraction coupling. However, in larger cells, a T-tubular system is necessary to allow transarcolemmal fluxes to occur deep within the cell interior. The presence of T-tubules compensates for the increased cell volume by providing a mechanism for overcoming diffusional restrictions by creating a much larger surface for ion fluxes from outside to inside the cell and vice versa. The T-tubular system is therefore an integral component of contraction and relaxation processes in mammalian myocytes. This is the site of highest density of calcium channels (for the influx of calcium) and the area where the sarcolemma is closest to the sarcoplasmic reticulum (the source of activator calcium for myocyte contraction; Figure 2-1).
In mammalian hearts, T-tubules are one of the last organelles to develop and generally do not appear until after birth. The time course of appearance of T-tubules varies among species (Table 2-1). The relative paucity of T-tubules limits the interaction between sarcolemmal calcium channels and sarcoplasmic reticulum calcium release channels, inhibiting the sarcoplasmic reticulum from participating in excitation-contraction coupling. Morphogenesis of T-tubules and the temporal relationship between the postnatal acquisition of T-tubules in the human heart and the emergence of a mature excitation-contraction coupling phenotype remain to be determined.
Coincident with the progressive development and maturation of the T-tubule system is a change in the appearance and function of the sarcoplasmic reticulum. The sarcoplasmic reticulum is a specialized form of endoplasmic reticulum that is an essential component of contraction and relaxation in the mammalian heart. In mature cardiac myocytes, the sarcoplasmic reticulum stores, releases, and reaccumulates the majority of the calcium that is involved in contraction and relaxation.
The sarcoplasmic reticulum is composed of junctional and longitudinal elements (the longitudinal sarcoplasmic reticulum is also referred to as the free sarcoplasmic reticulum). Important maturational changes in the amount, appearance, and function of the sarcoplasmic reticulum are apparent during late fetal and early postnatal maturation. In the adult, sarcoplasmic reticulum membranes are well organized and prominently related to the thick filaments of myofibrils. The junctional sarcoplasmic reticulum is immediately adjacent to the T-tubules in structures termed “dyads.” It is this close physical relationship that is the central element in the transduction of the changes in sarcolemmal membrane potential and calcium influx to the sarcoplasmic reticulum that trigger calcium release and contraction.
In mature myocytes, the junctional sarcoplasmic reticulum contains the sarcoplasmic reticulum calcium release channels. These channels (also known as ryanodine receptors because of their high-affinity binding to this neutral plant alkaloid), open during excitation-contraction coupling to allow discharge of stored calcium into the cytosol for contraction to occur. In addition, the junctional sarcoplasmic reticulum contains high concentrations of calsequestrin, a calcium-binding protein. This is the site of storage of most of the calcium that cycles through the sarcoplasmic reticulum to and from the contractile proteins with each sequence of contraction and relaxation. Triadin and junctin are smaller proteins contained in the junctional sarcoplasmic reticulum that are important in maintaining the proper spatial relations between calsequestrin and the calcium release channel. Other structural proteins include FK binding protein (also known as calstabin), which helps to stabilize the calcium release channels and promote coordinated calcium release.
Longitudinal sarcoplasmic reticulum contains the greatest density of the ATP-dependent calcium pumps involved in calcium reuptake into the sarcoplasmic reticulum. These proteins are termed “sarcoendoplasmic reticulum calcium ATPases” (SERCA). The cardiac-specific isoform is SERCA2a. The reuptake of calcium into the sarcoplasmic reticulum by SERCA2a is modulated by a regulatory protein, phospholamban, which is in close physical relationship with SERCA2a. In the basal unphosphorylated state, phospholamban acts as a “brake” to inhibit SERCA2a activity and calcium reuptake into the sarcoplasmic reticulum. When phospholamban is phosphorylated (eg, in response to β-adrenergic stimulation), the inhibition is removed, SERCA2a activity increases, and relaxation is facilitated due to enhanced sarcoplasmic reticulum calcium reuptake.
In the late fetus and early newborn, peripheral subsarcolemmal couplings between the junctional sarcoplasmic reticulum and sarcolemma can be observed prior to the acquisition of a well-organized T-tubular system. However, as the cells enlarge and the T-tubular system develops, deeper internal couplings (dyads) are acquired. The volume and distribution of the sarcoplasmic reticulum increase during late fetal and early postnatal maturation. Each of the components of the mature sarcoplasmic reticulum undergoes developmental regulation and maturation (ryanodine receptors, SERCA2a, phospholamban, etc.).
The sarcomere is the primary contractile unit of striated muscle (Figure 2-2). Contraction and relaxation of cardiac muscle depends on the structural organization of contractile and modulatory proteins into filaments that repeatedly move back and forth past one another. Anchoring of the structural filaments, combined with sliding of complementary filaments, results in shortening and generation of force. In mature cardiac cells, the myofibrils are organized into sarcomeres, which are delineated at each end by Z-discs. The Z-disc is a complex group of proteins that links the myofilaments from contiguous sarcomeres into a highly ordered network (see below).
FIGURE 2-2.
Schematic diagram showing arrangement of thick and thin filaments within the sarcomere. Thick filaments contain myosin, myosin-binding protein C, and titin. The tails of the myosin heavy chains are woven together to form the thick filament. The globular head projects outward to form cross bridges. The thin filaments include actin (which forms the backbone of the thin filament), the troponins, and tropomyosin. Tropomyosin binds to troponin T at multiple sites along the major groove of the actin filament and inhibits actin-myosin interaction. Troponin T binds the troponin complex to tropomyosin, troponin I inhibits interactions between actin and myosin, and troponin C binds calcium. The sarcomere, which lies between two Z-discs, is anchored by interactions between titin and actin with Z-disc proteins, including LIM domain-binding protein 3 (cipher/ZASP), α-actinin, cardiac LIM domain protein (MLP), and telethonin. Adapted from Mahony L. Development of myocardial structure and function. In: Allen HD, Driscoll DJ, Shaddy RE, Feltes TF, eds. Moss and Adams’ Heart Disease in Infants, Children and Adolescents, 7th ed. Philadelphia, PA: Lippincott Williams & Wilkins; 2008:577.

The Z-discs contain projections toward the center of the sarcomere that are termed “thin filaments” and contain an abundance of actin. Thick filaments are polymers of myosin and titin, a very large structural protein. The thick filaments are arranged along the same long axis of the sarcomere and are interspersed among the thin filaments (Figure 2-2). I-bands are composed of thin filaments, troponin complexes, and tropomyosin. A-bands are composed of overlapping thin and thick filaments. The dark M-band in the center of the A-band consists of thick filaments cross-linked to titin. Mutations in a variety of sarcomeric and Z-disc proteins are associated with both hypertrophic and dilated forms of familial cardiomyopathy (Chapter 9).
In early fetal life, sarcomeres can be observed in cardiac myocytes. However, the contractile apparatus remains relatively disorganized in the fetal and early newborn heart. Myofibrils initially are irregular and scattered about the interior of the cell. They are arranged in the subsarcolemmal region around a large central mass of nuclei and mitochondria in immature cells. As maturation progresses, the myofibrils come to lie along the long axis of the cell. Progressive organization of the sarcomeres occurs even as contraction and relaxation are occurring.
A progressive increase in myofilament content and maturation of the sarcomeres is consistent across mammalian species, although the timing may vary. A developmental increase in the myofibrillar population with a more orderly arrangement of myofilaments during maturation is often cited as the primary factor responsible for the increase in force generation observed during the late fetal and early newborn period in the mammalian heart. However, developmental changes in sarcomeric protein isoform expression also play an important role in maturational changes in force generation (see below).
Myofilaments are composed predominantly of myosin and actin (approximately 80% of the total contractile protein content). Other less abundant components, such as troponin and tropomyosin, provide regulatory control and structural support (Figure 2-2).
An essential component of the structural foundation of the sarcomere is titin, the largest protein known in humans. Titin spans half of the sarcomere from the Z-disc at the end to the M-line at the center. The N-terminal ends of titin overlap within the Z-disc, and the C-terminal ends overlap at the M-line to create a continuous filament system (so-called giant filament) that aligns the thick filaments within the myofibril.
Titin does more than simply connect the myofibrils. The segment of titin at the I-band contains serial spring elements that determine passive tension of the myocytes, thereby impacting late diastolic filling and resting sarcomere length. This likely contributes to the Frank Starling mechanism. In addition, this extensible region provides elastic recoil that drives early diastolic filling.
The predominant protein in thick filaments is myosin. Individual thick filaments contain several different polypeptides comprising a globular head and a tail section (Figure 2-2). Each of the approximately 300 myosin molecules in each sarcomere is composed of two heavy-chain myosin monomers and a stalk of four light-chain monomers. The heavy chains form a large, bilobed globular head and contain its ATPase activity, essential to release the energy necessary for cross-bridge formation. The light chains (essential and regulatory) form the long stalk of the thick filament, joined to the head by a flexible joint. It is the globular head that forms the cross bridge with the actin molecule, the ultimate step in sarcomere shortening.
An important protein associated with the thick filaments is myosin-binding protein C. This protein helps to stabilize thick filaments by forming transverse fibers that connect adjacent filaments near the center of the sarcomere. Other important structural proteins at the M-line include myomesin, M-protein, and obscurin, all of which appear to act with myosin-binding protein C to facilitate force transmission along the thick filaments.
Each thin filament contains three major proteins: actin, tropomyosin, and troponin (Figure 2-2). Repetitive association and dissociation of actin with myosin results in contraction and relaxation. Troponin is composed of three major subunits. The inhibitory subunit is troponin I (TnI), the calcium-binding subunit is troponin C (TnC), and the tropomyosin-binding subunit is troponin T (TnT). These proteins are required in the final stages of activation of actin binding with myosin to form the actomyosin complex, which initiates contraction.
Calcium plays an integral role in relaxation and contraction by binding to one of the troponin subunits, troponin C (TnC). Although there are several calcium-binding sites on TnC, attachment to the low-affinity site II is thought to be the trigger for cross-bridge formation. Binding of calcium to this site removes the inhibition that the tropomyosin-troponin complex confers on the actin-myosin interaction and triggers force generation and contraction. The number of cross bridges formed contributes to the amount of force developed by the contracting myocyte and depends on the amount of calcium released by the sarcoplasmic reticulum and intrinsic properties of the contractile proteins, such as calcium sensitivity. At any given calcium concentration, an increase in calcium sensitivity results in greater force and the rate of force generation. Conversely, a decrease in calcium sensitivity reduces force and the rate of force generation. Relaxation occurs as calcium dissociates from TnC and the proteins return to their resting conformational states.
Regulation of contraction and relaxation is very complex and cannot be explained simply by delivery of calcium to and removal from the contractile proteins. There is compelling evidence that cytosolic calcium peaks well before maximum force production is achieved and that calcium is nearly completely removed from the contractile elements before the onset of relaxation. These observations suggest that cooperative signaling and mechanical feedback through thick and thin filaments are critical determinants of contraction, force generation, and relaxation. In addition, a growing body of literature implicates posttranslational modification of sarcomeric proteins (phosphorylation, nitrosylation, and peroxidation) via various signaling pathways as important regulators of calcium sensitivity and cross-bridge cycling rates. The relationships among the various myofilament proteins, cytoskeletal proteins, extracellular matrix, and signal transduction pathways are complex and not fully understood. However, mutations or abnormalities in the function of many of these proteins clearly can have profound effects on cardiac contractile function. Mutations in myofilament proteins are associated with cardiomyopathy, which is more fully described in Chapter 9. As our understanding of intrinsic sarcomere function and regulation continues to expand, it is likely that new therapeutic approaches targeted at improving contractile function will be developed, but the implications for newborns with heart disease are not defined.
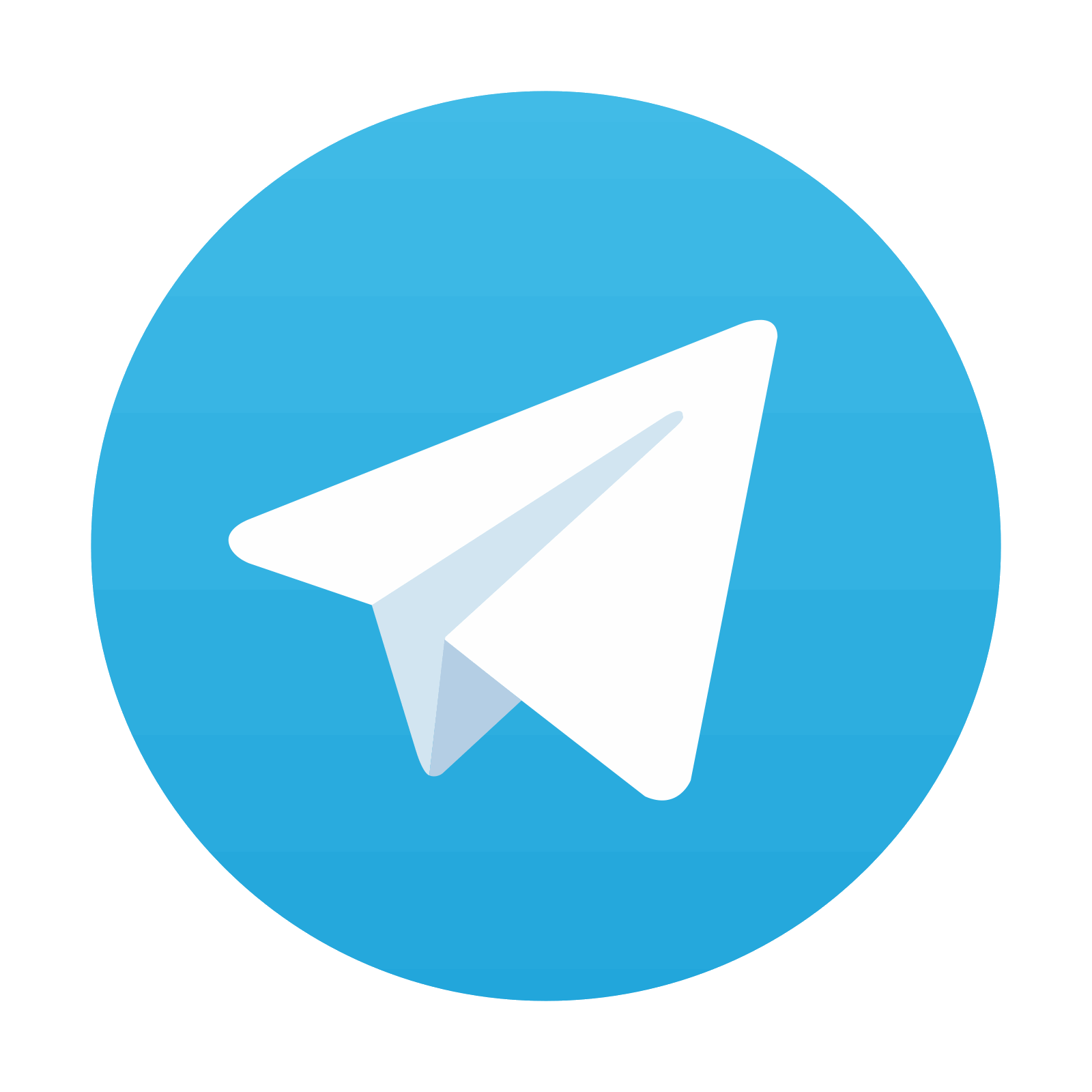
Stay updated, free articles. Join our Telegram channel
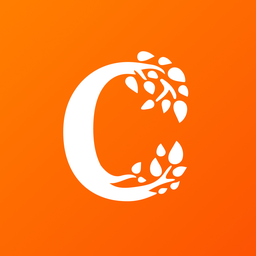
Full access? Get Clinical Tree
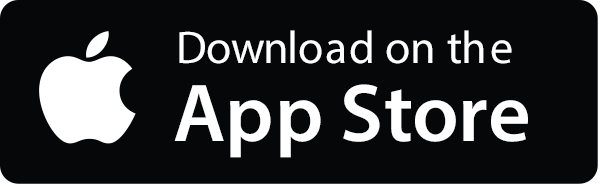
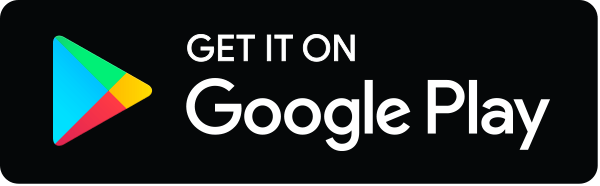