A functional myocardium is necessary for viability during embryonic and fetal development. As such, the heart is the first functional organ because its ability to distribute essential nutrients to the developing embryo is essential for viability and normal progression of development. In the human embryo, a beating heart is apparent by 22 to 25 days postcoitum, and continued development and viability depend on the heart’s ability to maintain circulation in the developing embryo, fetus, and for the rest of the organism’s life. Considering the necessity for vigorous cardiac function, it is not surprising that both congenital and acquired cardiac disease remain a major problem and is the most common cause of death in the adult population worldwide. This chapter reviews the origins of critical cardiac components and defines some of the basic components that underlie their function during development.
Early Myocardial Development
Different terminology exists regarding the timing of human embryologic development and terms, such as “conception,” “gestation,” and “pregnancy,” can lead to confusion that is further compounded by variations in defining the onset of development as occurring at the time of fertilization or implantation. Estimated gestational age is based on the time since the female’s last menstrual period (LMP) and is measured in weeks, but includes the roughly 14 days from the LMP to oocyte fertilization. Thus a woman who is 16 weeks pregnant based on her LMP is carrying a 14-week-old fetus. An alternative developmental measurement is the Carnegie staging (CS) system, which is widely used by embryologists to define embryo maturity. The CS system is based on external features of the embryo and ranges from CS 1 to CS 23, encompassing the time from postovulatory day 1 (CS 1) to approximately 53 to 58 days (CS 23) postovulation. In this chapter, cardiac embryology will be described primarily in terms of weeks postovulation with reference to CS. A comprehensive description of the morphogenic movements of the primordial heart progenitors is beyond the scope of this chapter, but the reader is referred to the many reviews as well as outstanding online resources (e.g., www.ncbi.nlm.nih.gov/pmc/articles/PMC1767747/ ) that detail these important processes.
Cardiac Looping
Structure underlies and ultimately determines function. Our understanding of the identity of the cells that populate and function in the heart, and their origins, has changed radically in the past 15 to 20 years. The generally accepted concept of an early heart tube containing all the necessary precursors of what will become a mature heart has been shattered by new technologies that allow the detailed dissection of cell lineages, sites of proliferation, and migration. Studies from the worm, fly, chicken, and mouse have enriched our understanding of human heart development as well, and we now know that many of the precursors for the various cell types in a mature heart are added to the primary heart tube at both the venous and arterial poles.
Molecular genetics has provided the tools to carry out detailed cell lineage tracings, showing clearly where the different precursors for the various components of the heart arise, how quickly they migrate, proliferate, and finally, when they express the specific proteins that serve as markers for the mature cell type. With the important exception of neural crest cells, which are derived from the neuroectoderm and contribute cells to the outflow tract and other, critical cardiac structures such as the valves and connective tissues as well as other organ systems, current data confirm that the heart is largely derived from the mesoderm. By 15 days a primitive streak containing cells that will migrate anteriorly is discernable ( Fig. 5.1 ). By 3 weeks, the human embryo contains a clearly recognizable structure populated by cardiac precursors located in the mesoderm, which is termed the cardiac crescent (see Fig. 5.1 ). The splanchnopleuric layer, which faces the endoderm, will give rise to the basic cardiac structures. The bilateral-located precursors fuse at the midline to form the cardiac crescent, and chick embryonic cells isolated from the same approximate developmental stage can be detected expressing proteins, such as cardiac-specific transcription factors, and a few sarcomeric proteins.

In human embryonic development, the cardiogenic region and primitive blood vessels of the embryo is present ~18 days postovulation (CS 7). Blood flow through the endocardial tubes and fusion of the two tubes into a midline structure is detectable at 20 days, with five recognizable segments of the heart tube (i.e., the truncus arteriosus, bulbus cordis, primitive ventricle, primitive atrium, and sinus venosus) at 22 days. Contraction results in the propulsion of blood from the sinus venosus to the truncus arteriosus. Looping of the primitive heart tube commences 23 days postovulation (CS 8). The primitive atrium, initially located caudally, moves cephalic and leftward of its original position while the future ventricular and outflow portions move caudally, ventrally, and rightward. Failure of correct rightward looping of the cephalad portions of the cardiac tube results in L-looping of the ventricles, where the morphologic right ventricle is located on the left side of the embryo and ultimately becomes the systemic cardiac pump. Cardiac looping is completed by day 28 (CS 10).
During CS 9, as the embryo folds, the linear heart tube continues to grow, partly through proliferation of the cardiomyocytes but mostly by adding newly differentiated cardiomyocytes, which are derived from the surrounding mesoderm at the poles. During this early stage of relatively restrained cardiomyocyte proliferation in the linear cardiac tube, cells that will make up the bulk of the heart are added from outside the primary tube along its entire length. The two groups of cardiac precursor cells are called the first and second heart fields (see Fig. 5.1 ). The first heart field gives rise primarily to the left ventricle and part of the atria with the right ventricle and outflow tract being derived from the second heart field. It should be noted that the outflow tract (see Fig. 5.1 ) and venous pole of the heart are both frequently involved in congenital heart defects. Therefore, understanding in detail the contribution and timing of the secondary heart field to cardiac development could shed considerable insight into the mechanisms underlying defects such as tetralogy of Fallot and DiGeorge syndrome. The migration and contributions of the primary and secondary heart field cells to the anatomy of the developing heart have been reviewed in detail, and are briefly summarized in Fig. 5.1 . Anatomically and functionally, the heart wall is normally divided into three layers with contractile function restricted to the myocardium, the middle layer of the heart wall, and flanked on the exterior by the epicardium, and the interior by the endocardium. The epicardium, vascular endothelium, and smooth muscle cells arise from the proepicardial organ (see Fig. 5.1 ), which exists transiently as an extracardiac cluster of cells arising as an outgrowth of the coelomic mesothelium at the ventrocaudal base of the developing heart. This process is considered in more detail in the “Fibroblast” section below.
Although briefly outlined in Fig. 5.1 , a complete description of the lineage tree for myocardial cells, their development, and the formation of the anatomic structures is beyond the scope of this chapter. For example, cardiac neural crest contributions are essential for formation of cardiac structures, such as the endocardial cushions (see Fig. 5.1 ) and the smooth muscle component of the pharyngeal arch arteries, and the reader is referred to several excellent reviews on these topics.
Atrial and Ventricular Septation
With the migration of the first and second heart fields completed, distinct delineation into atria and ventricles begins with the development of thin endocardial cushions at approximately 28 days (CS 10). The primitive cushions become increasingly filled with dense material as development continues. Atrial septation begins at 34 days postovulation (CS 14) with the appearance of a muscular shelf from the roof of the atrial component of the heart tube. This primary atrial septum shelf has a mesenchymal “cap” and grows between the systemic and pulmonary venous openings. The mesenchymal cap of the muscular shelf will eventually fuse with the superior endocardial cushions. The inferior portion of the atrial septum (the atrial spine) develops from mesenchyme approaching inferiorly from the posterior mediastinum, and likewise possesses a cap that will fuse with the primary muscular septum and subsequently with the superior and inferior endocardial cushions. These movements serve to close the primary atrial foramen (ostium primum), the gap between the leading edge of the primary septum and the endocardial cushions. The secondary atrial foramen (ostium secundum) is formed by the breakdown of tissue at the superior margin of the muscular primary septum. By 42 days (CS 18), the septum primum, septum secundum, and foramen ovale are formed.
Ventricular septation is both initiated and completed after the same activities in atrial septation, from 38 days (CS 16) to approximately 50 days (CS 22). The future left and right ventricular chambers (LV and RV, respectively) may be appreciated by 38 to 40 days, with the beginning of the muscular interventricular septum occurring concomitantly with the appearance of the apical portions of the LV and RV. By 42 days (CS 18), the muscular ventricular septum reaches from the floor of the ventricles toward the cardiac crux and the LV outlet portion is closed. At this point, the relatively large interventricular foramen allows communication between the ventricles and indeed is the connecting entrance to the RV. Ventricular septation is completed at approximately 50 days (CS 22) with closure of the inlet septum at the level of the atrioventricular valves.
Formation of Atrioventricular Valves
Following the initiation of endocardial cushion development at approximately 28 days, the atrioventricular canal becomes increasingly demarcated by the endocardial cushions, which are more apparent at 32 days postovulation (CS 13), although cellular content of the cushions is limited. Cushion density is increased by 34 days postovulation (CS 14), coincident with the fusion of the mesenchymal portions of the primary atrial septum with the superior atrioventricular cushion and the atrial spine with the inferior cushion. The atrioventricular junction can be seen at approximately 38 days (CS 16) with separate atrioventricular valves present by 42 days (CS 17 to 18). At this stage, the valve leaflets are still thick but will undergo remodeling to become thinner structures by approximately 56 days (CS 23).
Outflow Tract and Semilunar Valve Development
Outflow track septation and semilunar valve development begins with swelling of the truncal cushions at approximately 36 days postovulation (CS 15). Spiraling of aorticopulmonary septum is seen at 38 days (CS 16), with septation of the truncus arteriosus proceeding from distal to proximal. Development of the semilunar valves begins with endothelial to mesenchymal transformation of the endocardium to form cushions. Distinct but relatively thick semilunar valves are present at approximately 42 days (CS 17 to 18) and will eventually undergo extracellular matrix remodeling to become thin, stratified leaflets—a process that for mammals continues even after birth.
Comparison of Human and Mouse Cardiac Development
Zebrafish and chick models have been very useful in uncovering the lineages, the cell migration, and the anatomic timeline for cardiac development; they have the advantage of easily accessible structures that can be physically and molecularly manipulated. However, for a mammalian, a genetically accessible and experimentally affordable model system, the mouse heart most closely resembles the mature human heart and thus is widely used to investigate mechanisms of normal and perturbed cardiac development. Given the short gestation period of mice, the complex events involved in developing a four-chambered heart are understandably condensed. Thus events occurring over an approximately 4-week period in humans are accomplished in roughly 5 days in mice. The general order of events required to develop from an unlooped heart tube to a septated four chamber heart with four cardiac valves is the same in mouse and human, with atrial septation preceding ventricular septation and outflow tract development. In both species, development of the atrioventricular valves is a relatively long process. Because of the extensive use of the mouse heart in modern cardiovascular experimentation, a general comparison of the major events comprising human and mouse heart development is shown ( Fig. 5.2 ).

Origins of Structural Heart Disease
Many events in cardiac development occur simultaneously, resulting in common constellations of cardiac malformation. Truncus arteriosus occurs due to incomplete septation of the outflow tracts into a separate aorta and pulmonary artery from days 36 to 42 (CS 15 to 18), which in turn alters semilunar valve development and closure of the ventricular septum in the LV outflow region (days 38 to 42, CS 16 to 18). In some congenital heart defects, events early in cardiac developments do not necessarily affect subsequent events. For example, the genesis of “typical” congenitally corrected transposition of the great arteries (also referred to as L-TGA due to the leftward and anterior position of the aorta) is inappropriate looping of the primitive heart tube early in development (days 23 to 28, CS 8 to 10). This event results in a morphologic RV being situated on the left side of the body, and the morphologic LV residing on the right. Atrial situs is not affected, and the great arteries, while not having a normal anterior/posterior relationship, will nevertheless come to reside over the left-sided ventricle in the case of the aorta and over the right-sided ventricle for the pulmonary artery. As a result, the right-sided but morphologic LV will conduct desaturated systemic venous return to the low resistance pulmonary bed, and the left-sided but morphologic RV will conduct highly saturated pulmonary venous blood to the high resistance systemic circulation. While this is “corrected” circulation in the sense that the desaturated and saturated blood is routed into the appropriate vascular bed, when subjected to systemic pressure over the course of decades the morphologic RV is prone to dysfunction. In contrast, D-transposition of the great arteries is due to incomplete spiraling of the pulmonary and aortic trunks, resulting in connection of the aorta to the RV, and the pulmonary artery to the LV. Ventricular septal defects are commonly associated with D-transposition of the great arteries, consistent with the overlap in development of the ventricular outflow tract and ventricular septum. Many of these congenital defects have been elegantly modeled using sophisticated three- and four-dimensional renderings and are available online through mobile applications.
Major Cell Types in the Heart
Cardiomyocyte
When we think of the heart and then of the myocardium, the first cell type that comes to mind is the cardiomyocyte even though nonmyocyte cells constitute the majority of cells in terms of number. The cardiomyocytes form the muscular walls of the atria and ventricles, and are derived mainly from the mesodermal cells present in the first and second heart fields. By volume, cardiomyocytes are the predominant cell type in the heart. While it had previously been thought that they were the clear majority of cell type present, better technical methods for marking the distinct cell types have more recently determined that they account for between 25% and 35% of all cardiac cells. These highly specialized cells form the contractile basis of the heart and are filled with sarcomeres, the unitary contractile apparatus, as well as mitochondria, the Ca 2+ handling machinery and specialized protein-based macromolecular structures that can propagate rapid, directed, and unitary transmission of electrical signals among the cardiomyocytes, enabling the heart to function as a syncytium. Underlying these specialized functions, the cardiomyocyte is characterized by a well-defined spectrum of cardiac specific muscle proteins, including the cardiac myosins, cardiac actin, cardiac myosin binding protein C, phospholamban, various channel proteins, and others. The cardiac cardiomyocytes are further specialized, with unique cellular protein complements for the atrial and ventricular chambers, and distinct cardiomyocyte subtypes populating the heart’s conduction system. Atrial and ventricular cardiomyocytes have different contractile properties and electrophysiologic conduction profiles as well, making them highly specialized functional cells that occupy unique anatomic and functional niches in the heart. They are largely postmitotic and have limited regenerative capabilities. Although human cardiomyocytes are replaced at a low, but significant rate, regenerative capacity is clearly limited and cannot compensate when there is massive cell loss, such as occurs with a myocardial infarction.
Fibroblasts
The fibroblasts form the connective matrix of the myocardium. Cardiomyocyte health and function are inextricably linked to this supportive, extracellular matrix. Classically, the fibroblast has been rather ill-defined as a connective tissue cell derived from the primitive mesoderm. In situ in the myocardium, fibroblasts are visualized as small-sized, flattened cells that lack a basement membrane ( Fig. 5.3 ). They are literally tightly squeezed between, above, and below the cardiomyocytes, and they extend multiple cellular processes that form a dense network consistent with their main function, which in the healthy heart is to generate and maintain the extracellular matrix by producing the fibrillar collagens type I and II. This matrix is largely responsible for the three-dimensional architecture of the heart that is necessary for a normally functioning syncytium as well as fast and regular electrical activation. Considering its importance in both normal and pathogenic processes, it is striking how little we really know about the cardiac fibroblast. However, we are beginning to develop the tools necessary for its characterization in the form of relatively selective or specific markers that will allow lineage tracing and functional identification over time. We now know that prior to scar formation and beneficial or pathogenic remodeling processes, the fibroblast is activated to the myofibroblast, with concomitant transcriptional activation of a protein complement, including the matricellular protein periostin ( Fig. 5.4 ). Although the fibrotic process may be initially adaptive and provides the necessary regional strengthening, which is needed to preserve structural integrity in an injured myocardial wall, the myofibroblast population may continue to proliferate and differentiate, leading to additional scarring and pathogenic remodeling processes (see Figs. 5.3 and 5.4 ). The signaling pathways that underlie these processes and control both the activation and inactivation of myofibroblasts in response to injury are currently subject to intense investigation. While fibroblasts have often been thought of as being electrical “insulators,” the situation appears to be more nuanced. Current can be propagated, and specifically in the myofibroblast the cation-conducting channels are relatively insensitive to changes in voltage or remain open, resulting in a moderately polarized membrane (−20 to −40 mV). Improved optical and electrical recording technologies allowed conduction in cocultures of myofibroblasts and cardiomyocytes, confirming that heterocellular gap junctions developed. The electrophysiology of fibroblasts remains a field very much in flux.


The diverse cellular origins ascribed to the fibroblast population underlies and reflects the difficulty in defining cell markers that are absolutely restricted to fibroblasts. It is now generally accepted that the majority of these cells arise from the proepicardial organ (see Fig. 5.1 ), which subsequently serves as a source for the migratory cells that cover the developing heart and form the embryonic epicardial layer, and that contribute to the vascular endothelium. These epicardial cells support cardiomyocyte proliferation in the developing heart tube and provide the precursors for what will become fibroblasts and vascular smooth muscle cells. Some of these cells undergo an epithelial-to-mesenchymal transition, peel off from the epicardium and populate the atrial and ventricular walls, where, in the latter compartments, they are necessary for the formation of the compact myocardium. These cardiac fibroblasts continue to proliferate, essentially doubling in number during the postnatal period. They form the underlying myocardial scaffold and actively signal to and with the other cellular populations, including the cardiomyocytes, to actively proliferate at the appropriate developmental times or in times of cardiac stress.
Data defining the percentage of fibroblasts in the total cardiac cell population have varied widely with some investigators concluding that as many as 40% to 50% of the cells in the heart may be fibroblasts, although the majority of estimates place the percentages between 25% and 35%. Recent data from the mouse, using carefully defined, multiple markers and sophisticated lineage tracing techniques, have come up with surprisingly low percentages, with the fibroblasts comprising only approximately 20% of the nonmyocyte cells in the mouse heart or 12% to 15% of the total cells. These data have yet to be independently verified, but the investigators analyzed adult human cardiac tissue as well and those data mirrored the murine results.
Vascular Smooth Muscle Cells
These cells make up the main supportive cells for the vessel walls and regulate overall vascular tone in the heart (and elsewhere). Smooth muscle cells derive from a number of different mesodermal derivatives and sources, including the splanchnic, lateral plate and the somatic or paraxial mesoderm. Because of the complicated origins of these cells, and their inherent plasticity, there are conflicting data as to whether there are unique subpopulations. Smooth muscle cells can differentiate and then dedifferentiate as the vascular wall forms and remodels under different conditions. Embryonic smooth muscle proliferates rapidly and actively migrates as the nascent vasculature forms but cardiac vascularization is complex due to the multiple sources of the different cells that contribute to vascular development and the separate but related process of angiogenesis. Lineage tracing has shown that proepicardial cells (see Fig. 5.1 ) give rise to the smooth muscle cells found in the coronary arteries and epicardium. A prenatal vascular plexus arises from the epicardium, which is composed of a continuous layer of epithelial cells, some of which undergo an epithelial to mesenchymal transition to give rise, via vasculogenesis and angiogenesis, to the initial vascular network. However, lineage tracing studies showed that the postnatal vasculature may arise, in part, de novo from lineage conversion rather than mere expansion of the preexisting network.
The most important characteristic of the vascular smooth muscle cell (VSMC), which has been referred to as the engine of the vasculature, is its viscoelastic nature, allowing the cell to respond to deformation, load, stress development, and maintenance. As such, vessel tone can be modulated in response to internal and external environments. As is the case for the cardiomyocyte, where the myosin motor and its interaction with actin are essential for cellular function, actin and myosin interplay underlies VSMC function. Both of these proteins are represented by cell type specific isoforms, α-smooth muscle actin and smooth muscle myosin. Thus both cardiomyocytes and VSMCs depend on their respective molecular motors for normal and sustained contractile function.
VSMC proliferation/migration/accumulation is a determining factor in smooth muscle cell accumulation in the intima and thus is key to normal development as well as to pathogenic processes such as atherosclerosis. But, as our tools for lineage and cell tracing continue to improve ( Fig. 5.5 ), even this accepted dogma is coming under renewed scrutiny. Dedifferentiation and proliferation of mature smooth muscle cells, migration, and proliferation of stem and/or progenitor cells, or even resident stem cell populations in the vessel wall, are all under active investigation, highlighting the uncertainty of the origin and resident niches of VSMC progenitors.

Together with VSMCs, pericytes make up the so-called mural cells of the heart. This cell type, which is characteristically found in the terminal capillaries, is relatively underexplored in terms of its cardiovascular function, although its critical roles in maintaining and changing capillary circumference and impacting on coagulation in the brain are well documented. Pericytes are enveloped in the extracellular matrix and envelop the terminal endothelial-derived microvasculature in the heart. They have a star-shaped central body with long processes that enwrap the associated endothelial cells. Like VSMCs, they express α smooth muscle actin but at approximately one-tenth the level. The morphology and potential function of these cells in cardiac physiology have been recently reviewed. While some estimates for the population are as high as 10%, sophisticated lineage analyses indicate that approximately 6% of the cells present in the LV are mural cells.
Endothelial Cells
On the basis of endothelial cell-specific CD31 staining, analyses of healthy human hearts showed that endothelial cells make up 47% to 60% of total cardiac cells, and serve diverse roles. These cobblestone-shaped cells serve as protective plates in forming vessel walls, safeguarding blood transport, helping to control vascular permeability and, through signaling to VSMCs, regulating vascular tone. They are thus the defining cell type for the vascular system in both the heart and the general body plan. The endothelial cells that line the inner vessel walls are not inert structural entities, but rather actively signal to two major systems in the heart (and general body): the vascular and immune systems. Structurally, the endothelial cells comprise the lining of the vasculature and, for the larger vessels, are in intimate contact with the mural cells. Accordingly, they are a major cell mediator in maintaining hemostasis and their barrier function is critical as cell-cell junctions must be tightly controlled and quickly repaired in the event of trauma. In cooperation with platelets, the endothelium initiates the coagulation cascade responsible for blood clot formation that plugs the breach. Of course, when inappropriate coagulation occurs either temporally or topologically, the result can be fatal with the clot occluding a critical vessel conduit. At baseline then, endothelial cells are normally in an anticoagulant state but are highly responsive to both circulating factors and various procoagulants, such as von Willebrand factor and tissue factor, which are expressed in subendothelial areas on the extracellular matrix and become unmasked upon vessel injury.
Morphogenesis of the vasculature begins with the early appearance of the preendothelial cell, the angioblast in the mesoderm at CS 8 to CS 10. The angioblasts form cords both at their initial location and after migration to spatially separate sites they differentiate into the endothelial cells that form primordial cords or tubes. The heart tube is beating and a rudimentary circulation is apparent by CS 10, or 19 to 21 days postfertilization in the human. Development of the coronary arteries occurs around CS 16 to 18 and occurs in the subepicardial regions. A coronary plexus initially forms in the mesenchyme and subsequently remodels into the coronary artery system.
While the vasculature has been studied for over a century, there is a lack of understanding of the molecular influences that control its development, maintenance, and repair. Obviously, such an understanding would augment the ability to treat acute and chronic wound healing. As such, the potential pathways and mechanisms are being intensely investigated. Two distinct processes come into play. Vasculogenesis is normally considered to refer to the de novo formation of vascular channels and, as noted above, begins early during embryonic development as the free angioblasts coalesce into the loose cords. This process continues during and through organ formation. Angiogenesis refers to the processes that occur after formation of the primitive vasculature, as the early tubes spread and propagate, forming new networks into previously avascular regions through a process known as sprouting angiogenesis. This is accompanied by the splitting and fusion of the early tubes to form new connections and branches—a process called nonsprouting angiogenesis. Sprouting angiogenesis depends on active mitosis of the preexisting endothelial cells as well as their active migration, while nonsprouting angiogenesis often involves the splitting of a preexisting vessel via proliferation of the endothelial cells within the vessel wall and the subsequent expansion of the capillary bed. A number of pathways controlling these processes appear to be unique to the heart, and so extension of the various signaling pathways determined for other organ systems, or organisms may not be directly applicable.
Endothelial cells secrete the potent vasoconstrictor endothelin, a peptide with three isoforms. The predominant peptide, endothelin-1 (ET-1), is synthesized by the cell as a 39 amino acid peptide and processed by the membrane-based endothelin converting enzyme. The mature 21 amino acid ET-1 is released and binds to target receptors found on the adjacent vascular smooth muscle cells. ET-1 receptors are linked to the Gq and IP3 signal transduction pathways such that ET-1 release will also cause calcium levels to rise in the sarcoplasmic reticulum, increasing cardiomyocyte contractility as well. Endothelial cells also play a potent role in the innate immune response, and under inflammatory activation secrete the entire spectrum of toll-like receptors, which can trigger the proinflammatory cell response. This leads to structural changes of adhesion molecules that increase vascular permeability, secretion of inflammatory cytokines, the presentation of adhesion molecules that recruit leukocytes, and a switch to the procoagulant state.
Cardiac Regeneration
Can Cardiac Cells Regenerate?
As the above section makes abundantly clear, embryonic development is characterized by stem cell determination, the recruitment of cells from defined and, in some cases, multiple locations in the early embryo—but above all, hyperplasia of the determined cells in order to populate the developing heart. This is true for all four major cell types in the cardiac compartment: the cardiomyocytes, fibroblasts, mural cells, and endothelial cells. However, only the cardiomyocytes become postmitotic: the other three major cell types retain the ability to proliferate. Clearly, during embryonic and fetal development, and for a brief time after birth (a matter of days or, perhaps weeks in the human) there are increases in absolute cardiomyocyte numbers through both the proliferation of relatively immature cardiomyocytes and by the differentiation of precursor cells that have arisen through a stem cell population. Cardiomyocyte mass continues to increase as the heart grows and matures during postbirth development and maturation, but this is largely through physiologic hypertrophy, where the size of the individual cardiomyocytes increases as sarcomeres are laid down in series. Disease processes are often accompanied by changes in the proliferation of the different cell types; pathologic fibrosis occurs as a result of fibroblasts differentiating into myofibroblasts ; smooth muscle cell proliferation plays important contributory roles to vessel occlusion during the processes that underlie restenosis and atherosclerosis ; and proliferation of endothelial cells occurs as a result of vessel trauma and during arterial remodeling after myocardial infarction. Despite their regenerative capacity, cardiac cells do lose proliferative capacity as they age. For example, smooth muscle cell senescence has recently been associated with increased susceptibility to the development of atherosclerosis and plaque vulnerability, but the focus for augmenting cell renewal and proliferative capacity has appropriately been focused on the motor cell of the heart, the cardiomyocyte.
The regenerative capacity of the cardiomyocyte population is quite limited, and although it is not zero, it is clearly not sufficient to replace appreciable volumes of dead myocardium. Recently, a comprehensive study of human cardiomyocytes confirmed the early loss of regenerative capacity and showed good agreement with the majority of previous studies, which reported that less than 1% of the cardiomyocytes were replaced on a yearly basis in the adult heart. This lack of replacement capacity is critical under conditions where significant cardiomyocyte loss occurs, such as during and after myocardial infarction, in which up to 25% of the cardiomyocytes in the left ventricle (approximately half a billion) will die, and is in striking contrast to the other cardiac cell types. For this reason alone, the subject of cardiac regeneration has been intensely studied for over 100 years. A transformative observation in zebrafish lent new impetus to the search for a way to increase cardiomyocyte regeneration when Poss et al. showed that subsequent to partial (20%) surgical resection, a mature left ventricle could regenerate essentially all of its cardiomyocyte muscle mass. Although teleosts in general, and zebrafish in particular, can regenerate spinal cords, fins, and retinas as well, it remained for this cardiac regenerative capacity to be demonstrated in mammals. This was accomplished approximately 8 years later when Porello et al. showed similar regenerative capacity in mouse hearts. Importantly, innate cardiac regenerative capacity was quickly lost in the first week after birth. A critical observation was that the regenerative process is fueled by the proliferation of the preexisting cardiomyocytes and is not due to the recruitment and differentiation of a stem cell population or circulating, bone marrow-derived cells. However, data for cardiomyocyte regeneration and proliferation are riddled with contradictions and conflicting results, which is likely due to the wide variety of systems, strains, experimental technologies, and particular experimental contexts employed. For example, using genetic fate mapping in genetically engineered mice, Hsieh et al. showed that, after surgically induced cardiac injury through either transaortic constriction or myocardial infarct, the adult mouse heart could “refresh” its cardiomyocyte population through the recruitment of precursor cardiomyocytes or stem cells.
The existing consensus is that, under normal circumstances, cardiac regeneration and more specifically, cardiomyocyte turnover and replacement appears to occur very slowly, with less than 1% renewal on a yearly basis in the adult heart at the age of 20 years and less than half that rate after the age of 75 years. Although other investigators have reported much higher rates, these latter data could not be independently reproduced. From the cardiologist’s point of view, proof that any level of self-renewal is potentially transformative as it provides proof-of-principle that cardiomyocytes can be replaced. The current controversies and unanswered but pressing questions have been summarized in a recent Consensus Statement published by the American Heart Association.
The two seminal observations from the above discussion, that the early neonatal heart has significant regenerative capacity, and that even the adult heart retains some residual capacity, has led to an intensive search for the underlying mechanisms. The ultimate goal is to induce cardiomyocyte regenerative capacity therapeutically and thereby bypass the inherent difficulties in the exogenous introduction of cells into the heart. Increasingly, it has become possible to augment normal rates of cardiomyocyte renewal using exogenously delivered microRNAs to achieve regeneration by manipulating the cardiomyocyte’s genetic programming. Exposing the adult animal to gradual systemic hypoxemia over a period of a few weeks resulted in a significant augmentation of regenerative cardiomyocyte activity. Genetic manipulation of certain signaling pathways, such as the Hippo pathway in mice, also reactivated significant levels of cardiomyocyte regeneration. Other cell proliferative pathways and growth factors and their receptors also appear to be promising targets to positively modulate cardiomyocyte regenerative capacity.
To summarize, the last 10 years have been a stimulating decade of controversy and conflicting data, but consensus is beginning to emerge. We now know that the heart retains a small, but real cardiomyocyte regenerative capacity and that this capacity can be experimentally increased. Hopefully, this next decade will show us how to translate this endogenous capacity into effective therapeutic avenues in which injured myocardium can be efficiently and safely replaced, negating or diminishing the need for complex, exogenous, cell-based therapies.
Cardiomyocyte Development and Maintenance: From the Inside Out
A cell’s DNA is the basic template upon which its protein complement is written. However, it only represents the possible but incomplete template for the ultimate activity of the cell, as the protein population, functionality, levels, and locations are subject to hundreds or even thousands of internal and external modulating stimuli. In this section, we will explore the complexity of myocardial development in terms of the cardiomyocyte’s components—the goal being to understand how these mechanisms are reflected in the cell’s organization, organelles, and function under both normal conditions and during disease development.
Nucleus and DNA
Development, differentiation, maturation, and function all have their origins in the nucleic acid component present in the nucleus. However, the bulk of human DNA containing the approximately 18,000 to 20,000 genes is compressed through multiple interactions with discrete proteins, the nuclear matrix, and other chromatin components, rendering those sequences relatively inaccessible to both general and highly specific proteins that are necessary to initiate and maintain transcription. In most species, the cardiomyocyte is binucleated, with the genetic information in both nuclei capable of being transcribed. Although the general concepts remain largely unexplored in the heart, studies from a wide variety of cell types and organ systems have shown that the relative and absolute accessibilities of different genes are tightly controlled, beginning with the modulation of specific chromatin regions’ attachment points to the nuclear matrix.
The importance to overall cellular function, maintenance, and renewal of chromatin’s epigenetic status in general, and specific genes in particular, is becoming increasingly obvious. Particular DNA sequences can be chemically modified in a number of ways as modifying chemical groups are added to specific bases at specific locations. The role that increased cytosine methylation plays in silencing a particular gene locus is well documented, but the role that global DNA methylation plays in the development of coronary heart disease remains obscure. DNA base methylation, histone methylation and acetylation, as well as microRNAs and long noncoding RNAs (lncRNAs) are all epigenetic mechanisms that can play an active role in pathogenic cardiac processes such as fibrosis. Demethylating agents such as mocetinstat, a selective histone deacetylase inhibitor, can directly impact cardiac fibrosis. Cardiac hypertrophy is linked to histone methylation, as opposed to DNA methylation, and histone acetyltransferase activity results in acetylation of lysine residues in the histone’s tail region. This leads to chromatin decondensation and de novo accessibility of binding for bromodomain proteins and other transcriptional activators, promoting cardiac hypertrophy. As numerous signaling pathways and even small molecules or small nucleic acid analogues can dramatically impact the epigenetic status of a particular DNA sequence and thereby mediate its activation or silencing in cardiovascular disease, this is an emerging therapeutic approach that has not yet been convincingly translated in clinical cardiac practice.
RNA
Despite our long-standing knowledge of RNA editing and modifications, the field continues to surprise. N 6 -methyladenosine (m 6 A), a modification present in the 5′ “cap” structure and in other places along messenger RNAs (mRNAs) and lncRNAs, can be subsequently removed by the activity of RNA demethylases, giving rise to a new field called “epitranscriptomics.” There are now more than 150 different modifications known for mRNA but the functional significance of only a few are known. It is now clear that the overall translational efficiencies, splicing, export, stability, and other aspects of mRNA and lncRNA function and metabolism is regulated through the addition or removal of posttranscriptional modifications, similar to strategies used to regulate gene expression at the DNA and protein level. RNA posttranscriptional modifications are determined by the activity of “writer” complexes (methylase) and “eraser” proteins (RNA demethylase). The resultant changes in RNA structure and altered interactions between the modified RNA and other binding proteins or regulatory RNAs can have dramatic effects on the ultimate protein complement encoded by primary gene expression.
Co-transcriptional and posttranscriptional processing of mRNA is increasingly being recognized for the fundamental roles they play in determining or modulating the final protein complement. The concept that the basic posttranslational processes of capping the 5′ and polyadenylating the 3′ termini of the primary transcript is to control stability and translatability has been supplemented with a myriad of additional processes that determine the mRNA’s primary sequence and overall stability. The fundamental process of alternative splicing of the primary transcript, and its importance in determining the protein sequences that make up the cardiomyocyte’s critical protein complements have long been known to play a critical role in modulating muscle protein isoform content for many years, but understanding the macromolecular complexes that drive and control the processes has lagged. The exon-intron junction complex appears to underpin the structural scaffold upon which the protein components of the exon junction complex build during development, normal growth and maintenance, and in disease. For example, Pierrat et al. showed that two exon-intron junction complex proteins, eIF4A3 and mago nashi homologue protein, transit from the nucleus to the cytoplasm and back again in response to different metabolic stressors in cardiomyocytes. Modulation of the effective concentration of eIF4A3 in the nucleus using knockdown methods ablated all cardiomyocyte contractility within 96 hours in cardiomyocyte cultures, underscoring the potential importance of the control of this processing on maintenance of normal cardiac function.
Exon-intron junction selection has direct clinical implications as it dictates the primary structure of the mature protein. Understanding the mechanism is the first step in the rational design of drugs that can modulate the process. For example, mutations in the dystrophin gene are responsible for Duchenne muscular dystrophy (DMD), which affects striated muscle including the heart such that patients develop cardiomyopathy. The disease is discussed in detail later in this chapter. One relatively frequent (13% of DMD patients) mutation resides in exon 51. The mutation causes frameshifts, premature stop codons, and a lack of full length dystrophin expression, and an anti-sense-based stable oligonucleotide therapeutic, eteplirsen, has been developed that marginally increased the levels of stable dystrophin in clinical trials (4045-301 [ESSENCE]: phase III study; www.clinicaltrials.gov .). The mechanism of its action is shown in Fig. 5.6 . Although its efficacy is somewhat questionable as only minor increases in functional protein were measured, it cleared regulatory hurdles in 2016 and is being delivered to a small number of patients. Similar strategies are being developed for mutations that occur in other exons as well.

Protein Synthesis and Degradation
The relative stability of the perinatal population of cardiomyocytes highlights the importance of maintaining healthy proteostasis, particularly in this cell population. Early in life, normal growth of cardiomyocyte mass occurs not by proliferation, but by hypertrophy, indicating that protein synthesis must therefore be greater than protein degradation, such that the major contractile proteins accumulate and the cardiomyocytes lay down increasing numbers of sarcomeres. Classically, protein synthesis has been divided into three phases: initiation, elongation, and termination. Multiple controls exist for each, working separately and in concert to precisely control output from the preexisting mRNA pool.
Initiation occurs when a methionyl-tRNA, the 40S subunit of the ribosome and certain protein initiation factors form a complex that attaches to an mRNA and then scans along the 5′ untranslated region until it finds the initiator AUG (Met) that begins an authentic open reading frame. Recruitment of the 40S subunit to the correct AUG initiator codon involves recognition at the m7G “cap” located just upstream of the initiator methionine. The 5′-UTR can also contain additional elements that enhances scanning efficiency and increases translation off of a particular RNA. There are a number of diseases that are caused by mutations in the 5′-UTR. For example, a mutation in the stem-loop structure in the 5′-UTR of ferritin mRNA leads to hereditary hyperferritinemia by abrogating a mechanism that suppresses translation when the iron level is insufficient. Invariably 5′-UTR mutations lead to alterations in translation and pathogenic alterations to the cognate protein’s overall level. In an illustration of another mechanism, a C>T mutation in the internal ribosome entry site of the 5′-UTR of the c-Myc RNA allows cap-independent translation by facilitating the binding of nuclear ribonuclear proteins, such as HNPRK, resulting in enhanced translation and elevated protein levels. This mutation is present in a high proportion of patients suffering from multiple myeloma. Reduced translational efficiencies can also result from changes in the upstream nucleotides of the AUG initiator codon, and mutations in multiple proteins that act on initiation and/or elongation can lead to devastating pathologies.
Similar considerations and levels of control apply to the termination of protein synthesis, which is also precisely controlled, as is mRNA stability. Finally, protein stability, half-life, and degradation are each subject to multiple levels of control, and the mechanisms that govern protein levels can be exquisitely sensitive to various internal and external stimuli. Because of these multiple mechanisms, mRNA levels may often not reflect the protein level or, because of posttranslational modifications and protein activity. For these reasons, it is important to cautiously interpret pathogenic activity or causality on the sole basis of a polymerase chain reaction result, which only assays for a fragment of an RNA transcript. While polymerase chain reaction is clearly useful as a diagnostic tool when, for example, diagnosing viral myocarditis, the mere presence of high amounts of an mRNA may not reflect any increase in protein levels, due to posttranscriptional, posttranslational, or protein stability control mechanisms. The role these posttranscriptional and posttranslational modulatory processes can play was most clearly illustrated when it became possible to transgenically express proteins specifically in cardiomyocytes. The cardiomyocyte’s ability to maintain contractile isoform stoichiometry was tested by overexpressing the cDNA encoding the ventricular-specific myosin light chain isoform (MLC2V) in both the atria and ventricle. The promoter drove high levels of transgene expression in both cardiac compartments and was controlled in an appropriate manner during development. Ectopic overexpression of MLC2V led to compartment-specific replacement: the atrial-specific isoform (MLC2A) was essentially completely replaced although MLC2A RNA synthesis was unaffected ( Fig. 5.7 ). If the transgene encodes the isoform that is normally present (e.g., MLC2V expressed in the ventricle), the ventricle protein levels were unaffected, although the transgenic mRNA transcript accumulated to levels 5 to 10 times that normally seen. The transgenically encoded RNA was stable and was translated, indicating that the cardiomyocyte was able to maintain correct protein stoichiometry posttranslationally, presumably by more rapid turnover of the nascent, synthesized protein.

Protein and organelle turnover is critically important to overall development and cardiac homeostasis. During embryonic and fetal development, cellular protein must sometimes be rapidly turned over and the components recycled as new proteins for the new developmental stage are synthesized. It is particularly important for the cardiomyocyte as, in the absence of precisely controlled protein or organelle degradation, the cell would quickly become overwhelmed by damaged, misfolded, or fragmented proteins/organelles during its lifetime, with little prospect for rapid replacement due to the low regenerative capacity of this largely postmitotic cell population. A protein need not contain a loss- or gain-of-function mutation to be toxic. If the mutation results in a misfolded or unfolded protein that is not rapidly degraded, a protein conformation-based disease process can be initiated, leading to large, intracellular protein aggregates and inclusion bodies that by themselves can cause cardiac disease. Indeed, accumulation of these proteins in large aggregates is a hallmark of cardiac proteotoxic disease ( Fig. 5.8 ).

A series of diverse mechanisms are brought to bear on defective protein degradation. Chaperones are the first line of defense, participating in either the initial folding, or refolding of unfolded/misfolded proteins during or after protein synthesis. Indeed, preventing the accumulation of misfolded proteins begins during translation as chaperones attach to many nascent, growing peptides such that an estimated 30% of translated proteins never reach their final cellular destination. These newly synthesized nascent proteins are recognized as being misfolded or are unable to be folded correctly and are degraded almost immediately after or even during translation. Heat shock proteins are perhaps the most intensely studied chaperones, and their importance in cardiac function is well documented. However, chaperones are only a part of the multifaceted protein quality control machinery, which consists not only of constitutive and inducible chaperones but also engages compartment-specific mechanisms such as the unfolded protein response pathways in the endoplasmic reticulum or the heat shock response in the nuclear and cytoplasmic compartments.
The endoplasmic reticulum (ER) stress response, also referred to as the unfolded protein response, is another important protein quality control mechanism in the heart. Three transmembrane ER proteins (PERK, IRE1 and ATF6) are activated by misfolded proteins in the ER, stimulating the translocation of transcription factors ATF4, ATF6, and XBP1. This then activates proteins that comprise the ER stress response, including chaperones, targeting the misfolded proteins for degradation by the proteasome (see below). Unsurprisingly, ER stress is induced with cardiac injury including ischemia, pathologic hypertrophy, and heart failure.
However, the main system of defense for ridding the cardiomyocyte of damaged or misfolded protein is the ubiquitin proteasome system. This system mediates the turnover of most cellular proteins by degrading terminally misfolded proteins via proteasomal proteolysis. The proteasome is a large, multisubunit complex tasked with the degradation of monomeric proteins and the majority of misfolded protein species are eliminated from the cell using this mechanism. For proteasomal degradation, the proteins must first be posttranslationally modified by the process of ubiquitination. Ubiquitination involves three main steps: activation, conjugation, and ligation, which take place through the sequential actions of a series of reactions catalyzed by the E1, E2, and E3 enzymes, respectively. The damaged protein is first covalently conjugated to a series of ubiquitin monomers at specific lysine residues through an isopeptide bond, serine or threonine residues via an ester linkage, or at the N-terminus through a peptide bond with the amino group of the methionine. Through multiple catalytic rounds, successive ubiquitins are linked to the attached (growing) chain at either one of seven lysines or the terminal methionine, growing into a poly-ubiquitin chain, although mono-ubiquitination sometimes occurs. The modified protein is then recognized by transport proteins and trafficked to the proteasome for subsequent proteolysis. The proteasome is equipped with proteases, which have cleavage activities similar to trypsin, chymotrypsin, and caspases that enable most proteins to be degraded within the proteasomal cavity or bore. The fidelity of this process is critical; malfunction or decreased proteasomal proteolysis will lead to misfolded protein accumulation and cellular dysfunction.
Interestingly, proteasomal activity is impaired in a number of heart failure models, and proteasomal activity is a therapeutic target in treatment of different blood cancers, with inhibitors of the 20S proteasome, such as bortezomib or marizomib, validated in clinical trials. There has been interest in enhancing proteasomal activity and subsequently ascertaining if cardiac function is maintained in the face of an acute or chronic proteotoxic insult. In a series of experiments utilizing crosses between different transgenic mice, Wang and colleagues showed that enhancement of the proteolytic function of the proteasome was beneficial in cardiac protein conformation-based disease or in ischemia-reperfusion injury, although clearly in some contexts of cardiac disease, increased proteasomal flux can be detrimental.
The proteasome’s activity is dependent on the targeted protein being internalized into the catalytic bore of the cylinder formed by the proteasomal protein complex. This imposes size limits, as the bore’s internal diameter is, at most, 53 Angstroms. However, during proteotoxic stress, large proteinaceous aggregates or cell organelle damage occurs, resulting in cellular debris too large to be degraded by the proteasome. When this occurs, the quality control mechanism autophagy comes into play.
Autophagy (“self-eating”) refers to a cell’s ability to recycle proteins and damaged organelles. It is a normal process that is essential during normal development and maturation, as well as for normal maintenance of cell function Autophagy can serve many purposes and its modulation, either up or down, may be beneficial or pathogenic, depending on the cellular context, underlying disease, and developmental stage. However, in the face of a proteotoxic insult in the heart, autophagy can serve as the primary clearance mechanism for proteinaceous aggregates or even damaged mitochondria and other organelles too large for proteasomal degradation. Macroautophagy involves the engulfment of the components destined for degradation into double-membrane vesicles known as autophagosomes, which fuse with lysosomes or endosomes to promote degradation. Typical cargo degraded during autophagy includes misfolded proteins and defective organelles. Because autophagosomes can accommodate much larger cargo than the proteasome, they serve as the primary degradation route for these large damaged cellular components. Compromised autophagy, in the context of proteotoxic heart disease, presents an increased pathogenic insult and can lead to increased morbidity and accelerated heart failure. Decreased autophagic flux has been noted in many disease processes but autophagy is very context dependent and restoration of normal or even enhanced autophagy can be beneficial or pathogenic.
Contractility and Development-Specialized Machinery of the Cardiomyocyte
Sarcomere
The sarcomere is the basic, force producing component of striated muscle and tightly ordered arrays of these micrometer-sized machines are the hallmark ultrastructural anatomy and defining characteristic of the cardiomyocyte. As such the sarcomeres must be assembled before contraction begins in the developing heart. Sarcomeric structure and function are dominated by three filament systems ( Fig. 5.9 ):
- 1.
The thick filament, which consists predominantly of myosin and its associated light chains.
- 2.
The thin filament, containing long chains of polymerized actin. Other thin filament proteins include tropomyosin, which rests proximal to the groove in the actin filament, and the troponin complex, which, together with tropomyosin (see Fig. 5.9A ), forms the switch that allows productive (force-generating) binding between the thick and thin filaments and contraction, followed by relaxation. This “sliding filament” model underlies all aspects of cardiac mechanics and the ability of the developing and mature heart to pump.
- 3.
The titin filament, a giant protein that forms the underlying scaffold for the sarcomere unit. Each titin molecule spans one-half of a sarcomere with the amino terminus at the Z disk and the carboxy terminus at the center M line (see Fig. 5.9A ).

However, it is somewhat misleading to think of the sarcomere as a simple unit consisting of only a few proteins as there is an ever-growing list of essential proteins that interact at and with the different filament systems, many of which display multiple isoforms that are developmental-stage and/or muscle-type specific. Multiple mutations exist in many of these proteins and are responsible for a large proportion of congenital heart disease as well. In fact, well over a thousand mutations in multiple sarcomeric proteins have been associated with human cardiac disease. Mutations in the myosin heavy and light chains, actin, tropomyosin, the troponins, myosin binding protein C, titin, α-actinin, and ~15 other proteins associated with the sarcomere have given rise to thinking about hypertrophic cardiomyopathies as “disease(s) of the sarcomere.”
Myosin
The thick filament consists mostly of myosin by mass. Considering its central importance to cardiac function, it is not surprising that myosin has been a focus of intense study since the 19th century. It is the single most abundant muscle protein by mass, constituting greater than 50% of myofibrillar mass and 15% to 30% of total muscle protein. Myosin is a hexameric protein made up of two heavy chains (Mr; 229,000) and four light chains (Mr; 18,000 to 27,000) (see Fig. 5.9A ). The heavy chains consist of two separate domains; a globular head region and a rod region that assumes an α-helical coiled coil. The adenosine triphosphatase (ATPase) activity underlying muscle contraction is localized at the amino-terminal end, which corresponds to the globular head and neck of the molecule. High-resolution x-ray crystal structures of the head region have provided significant insights into the functionally important domains of the molecule. The binding sites for actin and ATP are located in the globular head, whereas the myosin light chains lie just distal to the head, in the so-called neck or hinge region. The two types of myosin light chains, essential and regulatory, likely have structural and regulatory roles in cardiac muscle contraction. As is the case for many muscle proteins that underlie the physiologic requirements and contractile properties of different muscles, there exist particular myosin isoforms in cardiac muscle, termed α- and β-myosin heavy chains, which are encoded by MYH6 and MYHC7 , respectively. Although the two isoforms share greater than 90% homology, their enzymatic activities differ significantly, leading to specific profiles in rates of shortening, development of power, and force, and differences in the metabolic economies of force generation muscle energetics. The relative amounts of the different isoforms vary depending on the developmental stage, the particular region of the heart, and the animal. The two heavy chain genes MYH6 and MYHC7 give rise to the two unique homodimers, V1 and V3, respectively. An intermediate heterodimeric form, V2, can also be detected. Each of these forms displays different electrophoretic mobilities in polyacrylamide under nondenaturing conditions, and each has a different intrinsic ATPase activity; V1 is the most active (by about three- to fourfold) and V3 the least. The isoform population correlates with the different intrinsic muscle shortening velocities observed between species and within a species as the heart responds to chronic stress or conditioning. The preponderance of evidence suggests that the adult human ventricle, contains essentially only V3. Extensive circumstantial and causative data support the hypothesis that the speed of contraction is dependent on the myosin isoform present. Studies have confirmed that the sliding velocity of the myosin-actin complex correlates with the particular myosin heavy chain isoform. Using rodent models, Alpert and associates noted early on that myosin’s ATPase activity is lower in the failing heart and postulated that molecular changes in the protein (later confirmed to be isoform shifts) play a major role in functional adaptation of the hypertrophied heart to chronic overload. During this process, α-myosin, which has a relatively high ATPase activity, is downregulated, and the synthesis of β-myosin increases. The resultant lower ATPase activity presumably mediates a negative inotropic effect resulting in reduced myocardial contractility by decreasing the cross-bridge cycling rate. However, systolic ejection is increased because of increased tension. By carefully measuring the energetics, Alpert’s group showed that there is an overall increase in the efficient use of chemical energy to generate mechanical force as a result of the isoform switch.
While it is generally accepted that the isoform shifts result in different functional endpoints, until recently it was thought that the human ventricle did not undergo such a transition: the myosin is and remains in the adult, V3. However, an isoform shift can occur in the human ventricle, at least at the transcriptional level, with a mixture of myosin isoforms present. In the left ventricle significant down-regulation (by 67% to 84%) of β-myosin accompanied development of congestive heart failure. Subsequently, Nakao et al. were able to carefully quantitate the relative levels of the transcripts encoding the two isoforms in ventricular free wall samples derived from donors with no history of cardiovascular disease and compare those data with the ratios found in samples of LV free wall obtained from 19 patients undergoing transplant for end-stage heart failure. They found significantly elevated levels of α-myosin mRNA of about 30% in the healthy hearts compared to only 2% in the diseased ventricles. However, a subsequent study was unable to reproduce those data.
In light of these data, it was exciting when mutations in human β-myosin were first identified as being responsible for familial hypertrophic cardiomyopathy. Both gene targeting and transgenic approaches were quickly directed at making the murine models. Dozens of different mutations in β-myosin are now linked to familial hypertrophic cardiomyopathy, but one of the most severe is Arg403Gln. This residue is contained within the globular head region, and crystallographic data indicate that it is close to the actin-myosin interface. The effects of the mutation were analyzed in vitro and showed that the protein had normal ATPase activity in the absence of actin, but the V max of the actin-activated ATPase was reduced.
Actin and Titin
The thin (actin-containing) filaments, consisting of coiled coils of polymerized actin are anchored firmly in the Z disks, which form the boundaries of the single sarcomere. Actin filaments have polarity, containing both pointed and barbed ends each of which is capped by the proteins tropomodulin and CapZ (at the Z-disk), respectively. The thin filament extends from each Z-disk toward the center of the sarcomere. At their Z-disk termini they do not interact with the myosins and hence form the so-called “I-bands” (isotropic under polarized light) (see Fig. 5.9 ). In the “A” (anisotropic)-band, they are interdigitated with the myosin thick filaments. During muscle contraction, the actins slide past the myosin, bringing the Z-disks at the opposing ends of the sarcomere closer to one another. Missense mutations in different regions of the actin can result in either dilated cardiomyopathy and heart failure or familial hypertrophic cardiomyopathy. The actin filaments have polarity containing both pointed and barbed ends, each of which is capped by the proteins tropomodulin and CapZ (at the Z-disk), respectively.
While it is the myosins in the A-band that are responsible for transducing chemical energy into mechanical force, the I-band links those machines to the Z-disk boundaries, allowing the sarcomere to shorten. The I-band also contains a portion of the third filament system, titin, in which two giant titin molecules, each one attached to the Z-disk extend a half-sarcomere length to the sarcomere’s center. Both the actin and titin filaments are firmly anchored at the sarcomere Z-disk boundaries, providing firm attachments at which shortening force can be transduced into effective movement of the overall sarcomere as well as providing the spring-like resilience needed for repeated cycles of shortening and relengthening inherent to sarcomere function. I-band titin sequences also are a key determinant of passive force generation and determine passive tension during sarcomere extension. I-band titin sequences are variable and can be altered by extensive alternative splicing and posttranslational modification, underlying its responsiveness to stress-induced signaling pathway-alterations. The extensible region in the I-band (shown in Fig. 5.9A ) thereby is a key influence in determining overall diastolic function. Numerous titin mutations have been confirmed as causative for both cardiac hypertrophy and dilated cardiomyopathy.
Extensive data obtained from a wide variety of small and larger animals, such as the mouse, rat, guinea pig, and rabbit, show that the relative levels of sarcomere protein isoforms are modulated during development and various disease processes. Isoform changes, in turn, can effect significant changes in cardiac hemodynamics. These considerations are not restricted to the motor proteins but rather are recapitulated across a spectrum of proteins that are both intrinsic components and activating partners of the sarcomere that modulate sarcomeric activity and sensitivity to external stimuli. The ancillary myosin light chains also exist as isoforms that are both compartment specific and are modulated during disease. The sarcomeric regulatory proteins, such as troponin and tropomyosin, also exist as isoforms whose ratios can change during development and disease. However, the physiologic significance of many of these isoform shifts has not been rigorously established. A closer examination of the trimeric cardiac troponin, is informative and illustrates how modulation of a sarcomeric component can affect overall contractile function.
Cardiac Troponin
The troponin complex consists of a Ca 2+ binding protein termed troponin C (TnC), a tropomyosin binding subunit, TnT and the so-called inhibitory subunit TnI. Together, the complex serves as a Ca 2+ -sensitive “molecular switch” of striated muscle contraction.
In both the rat and human hearts, TnI isoforms are developmentally regulated, with the fetal heart expressing proteins encoded by TNNI1 (slow skeletal muscle gene or ssTnI), and TNNI3 (cardiac (c)TnI). During development and maturation, ssTnI gradually decreases such that the adult heart expresses only cTnI. The two isoforms have different Ca 2+ sensitivity profiles and different pH optima as well, which has important consequences for protection against acidosis in the immature heart, as acidosis in cardiac muscle leads to decreased force development. ssTnI is much more refractory to this effect than cTnI and thus decreases the sensitivity of myocardial force production to acidosis. Systemic elevation of TnI due to cardiomyocyte leakage as sarcolemmal integrity is lost is a widely used biomarker of myocardial damage.
TnT, the tropomyosin binding subunit, is represented in the human genome by three distinct sequences encoding a slow skeletal isoform ( TNNT1 ), a fast skeletal TnT ( TNNT3 ) and the cardiac TnT encoded by TNNT2 . Each of these primary transcripts can undergo alternative splicing, generating a large array of different TnT isoforms, which are referred to as numbered isoforms (e.g., cTnT1, 2, 3…n) whose unique functions remain incompletely cataloged but which are differentially expressed in normal and diseased hearts. The cTnT1 and cTnT2 isoforms are both expressed during cardiac development but soon after birth cTnT1 levels decrease and cTnT3 appears to be the only isoform in the normal adult heart. The cTnT4 isoform is also expressed during development but is not normally found in the adult. However, re-expression can be detected in the failing adult heart.
TnC, which is a calmodulin-related protein containing EF-hands at each termini, binds Ca 2+ and forms the third part of the troponin switch. The inhibitory subunit, TnI by itself completely inhibits actomyosin ATPase activity in vitro. TnT binds to tropomyosin, a protein consisting of 284 amino acids, which assumes a highly extended parallel coiled-coil that lays along the actin filament (see Fig. 5.9A ). When Ca 2+ binds to TnC, a conformational change takes place such that TnI’s conformation is also modified, allowing TnT to interact with tropomyosin such that the entire tropomyosin–troponin complex is shifted. This results in strong binding between the actin and myosin filaments, allowing force production to occur, as ATP is split by myosin’s ATPase activity, producing a conformational change in the myosin head that results in the directed translation of the actin filament relative to the myosin thick filament. Relaxation depends on release of the bound adenosine diphosphatese–myosin complex from actin, which depends on attachment of a fresh ATP at its binding site in myosin’s head region, as well as Ca 2+ depletion. On depletion of Ca 2+ TnC no longer can bind to the ion, allowing TnI to switch back to its inhibitory conformation and tropomyosin assumes the steric hindrance that prevents strong crossbridge formation between the thick and thin filaments.
As alluded to above, during development, variable isoforms of the three troponins are expressed. Cardiac TnI and TnC isoforms arise from different genes but cTnT isoforms are generated via variable splicing patterns that appear to be developmentally regulated. However, the functional consequences of these isoform shifts have not been fully determined. Presumably, they play a role in modulating shifting calcium sensitivities of sarcomere activation during cardiomyocyte maturation. Ca 2+ sensitivity of the molecular switch can also be modulated by posttranslational modifications, particularly phosphorylation of TnI by protein kinase A, which induces an increase in the rate of crossbridge cycling. Although Ca 2+ cycling through sequestration and release from the sarcoplasmic reticulum is usually considered to be the major determinant in the crossbridge cycling rate, the internal components of the sarcomere are capable of significantly altering the kinetics of force production and relaxation via posttranslational modification of both the thick (e.g., myosin, cardiac myosin binding protein C) and thin filaments (the troponin complex) are capable of significantly altering the kinetics of force production and relaxation. A number of recent excellent reviews cover these topics in detail.
We have restricted the discussion to a few sarcomeric proteins to illustrate the potential species of contractile proteins present in the sarcomere, but the picture is necessarily incomplete considering the number of proteins and the posttranslational modifications protein(s) can undergo, which can dramatically affect the sensitivity, duration, and force production of the contractile apparatus. The troponins, myosins, cardiac myosin binding protein C, titin, and others are all subject to multiple posttranslational modifications, such as phosphorylation, acetylation, SUMOyation, etc., each of which can significantly affect protein stability, trafficking, overall activity, and myocardial ability to maintain or even augment cardiac output. It is clear that force production is affected during development and by disease processes, and can be modulated by changing the activities of different contractile proteins through either isoform substitutions or posttranslational modifications. Compromised activity can lead to cardiomyopathy and/or decreased cardiac contractility resulting in systolic heart failure.
Current heart failure therapies largely rely on blocking neurohormonal activation. However, the basic motor unit remains an attractive but refractory therapeutic target. By screening a small molecule library for compounds that increased myosin ATPase activity, Morgan and colleagues identified a compound that effectively activated the β-myosin heavy chain’s enzymatic activity. After slight modifications to improve its pharmacokinetic profile and specificity, the compound was named omecamtiv mecarbil and successfully tested in phase I and II clinical trials. In subsequent studies, it was shown that the drug increased ATPase activity by accelerating the actin-dependent rate of inorganic phosphate release from its bound position on the myosin head. This activity appears to be restricted to the cardiac isoform. The drug thus represents a fundamentally different approach from existing drugs for augmenting contractility. In 2016, a phase III cardiovascular outcomes clinical trial of omecamtiv mecarbil, GALACTIC-HF, was announced with a goal of enrolling 8000 chronic heart failure (LV ejection fraction [LVEF] ≤35%) patients in 34 countries. The primary endpoint is a composite of time to cardiovascular death or first heart failure event, which is defined as either a hospitalization for heart failure or other treatment for worsening heart failure ( ClinicalTrials.gov identifier: NCT02929329).
Mitochondria and Energy Production
Mitochondria are the powerhouses of the eukaryotic cell. Derived from ancient, prokaryotic symbiotes, mitochondria are unique in that they contain their own distinct genome (so named mitochondrial DNA, or mtDNA) that is transmitted exclusively through the female germline. The double-stranded circular mtDNA consists of 16,569 nucleotides encoding 37 genes. These are transcribed into two ribosomal RNAs (rRNA), 22 transfer (t)RNAs and 13 mRNAs that are translated into 13 unique proteins, which are distributed amongst the respiratory complexes I, III, IV, and V. The two ribosomal RNAs and 22 tRNAs encoded by the mtDNA are essential for the mitochondrial translational machinery used to generate the 13 polypeptides. All 37 genes are essential but not sufficient for normal mitochondrial function. Indeed, the bulk of the approximately 1000 proteins that make up mitochondria are encoded by nuclear genes, and the interplay between the transcription and the translation of the nuclear-encoded and mitochondrial-encoded sequences is tightly controlled.
An adult human heart can consume 6 kilograms of ATP daily. At any one time, the heart contains about 700 mg of ATP, which is sufficient to sustain pump activity for only about 10 seconds (at 60 beats/min). The normal heart is able to manufacture, de novo, more than 95% of this amount through the process of oxidative phosphorylation. Utilizing the tightly integrated activities of four respiratory enzyme complexes, a proton gradient across the mitochondrial inner membrane is produced and maintained, thereby providing the energy to drive the membrane-bound ATP synthase. Any compromise in the overall health of the mitochondrial population will invariably lead to cell stress, dysfunction and even death. In fact, mitochondria are often referred to as the “gateway to apoptosis” or programmed cell death. Apoptosis can be triggered simply by impairing the electron transport chain or mitochondrial membrane potential, leading to the release of mitochondrial stores of cytochrome c and activating caspases that initiate apoptosis.
Mitochondria are normally restricted to well-ordered arrays, in which the mitochondria are positioned directly over the A-bands and I-bands containing the energetically demanding contractile apparatus ( Fig. 5.10A ). The architecture is not spontaneously formed but rather reflects the dynamism of mitochondrial trafficking. Mitochondria can be coupled to multiple dynein or kinesin motors and transported, depending on the motor to which they are attached, in either direction along the microtubule, cytoskeletal actin or desmin networks of the cell’s cytoskeleton. They are then tightly enmeshed in the desmin intermediate filament network so that they remain in close proximity to the sarcomeres. If these transport mechanisms or the intermediate filaments are disrupted, mitochondrial distribution becomes aberrant (see Fig. 5.10B ) and the energy supply fueling the contractile cycle can become disrupted, leading to decreased myocardial power output of the myocardium. Mitochondria can also display active membrane remodeling processes, resulting in either fission, by which means they reproduce and multiply, or fusion that in some cell types can result in a single, interconnected mitochondrial network that is tightly controlled and integrated. These opposing processes must be balanced and are responsive to stimuli that can activate or inhibit mitochondrial biogenesis or fusion. In cardiomyocytes, the appearance of numerous, small mitochondria that are not tightly juxtaposed against the well-ordered sarcomeric arrays can be a sign of stress and/or an attempt to repopulate a compromised mitochondrial population (see Fig. 5.10B ).
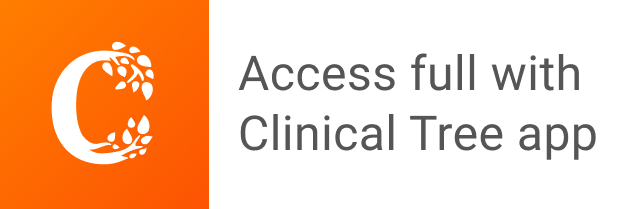