The developing heart appears to be simply a smaller version of the adult heart, based on global function. The ventricles of the adult and the developing heart fill with blood, develop pressure, eject blood, and relax ( Fig. 4-1 ). Across development, nonetheless, ventricular myocardium differs quantitatively and qualitatively in function and structure. For example, adult myocardium develops greater active tension than does fetal myocardium, and is more compliant 1 ( Fig. 4-2 ). When the extracellular matrix that enfolds the cells is removed, a marked developmental increase in contractility is observed in the isolated cell. The velocity and amount of sarcomeric shortening of the adult myocyte are greater than those of the immature myocyte 2 ( Fig. 4-3 ). In this chapter, I review the structures and processes that are basic to cardiac function, and show how they are affected by development.



THE HEART
The volume and mass of the heart increase with development. 3,4 The postnatal increase in left ventricular mass in the structurally normal heart is a result of an increase in the number, or hyperplasia, and in size, or physiological hypertrophy, of the ventricular myocytes, and in the growth of the non-myocytic components of the myocardium. 5 Workload and mural stress directly affect mural thickness, as evidenced by the differences in thickness of the right and left ventricular free walls of the adult heart. With birth, the relative workloads of the ventricles change, with that of the left ventricle being increased. This postnatal change in workload is associated with an increase in left ventricular mass, relative to body weight, while that of the right ventricle remains the same or decreases. 4 The ability of the developing heart to increase ventricular mass in response to an increase in mural stress is exemplified by changes in the left ventricle of the newborn with concordant atrioventricular and discordant ventriculo-arterial connections. The normal postnatal increase in left ventricular mural mass does not occur in the presence of the normal neonatal fall in pulmonary arterial pressure. If, however, the pulmonary arterial pressure is elevated in the infant through surgical constriction of the discordantly connected pulmonary trunk, left ventricular mass increases markedly within a few days.
Hyperplasia
During fetal and early neonatal life, division of cells is the primary mechanism by which myocardial mass increases. 3,4,6 In response to the greater workload borne by the postnatal left ventricle, the population of myocytes increases during neonatal life more rapidly in than in the right. Of note, in the mammal, hyperplasia, or the process of cytokinesis, is thought to cease after the first month or so of neonatal life. Evidence for hyperplasia in the adult heart has been provided in the adult amphibian heart, nonetheless, and more recently in the mammalian heart. The extent to which these mechanisms, which may involve resident cardiac stem cells in the adult, generate additional myocytes in the adult mammalian heart remains to be established. 7–9 The potential for division of myocytes, or generation of myocytes from stem cells, and the mechanisms underlying this process are, at present, topics of great interest and debate because of the need to develop new therapies for the failing heart. 10,11
Hypertrophy
Increasing size of the cardiac myocytes, or physiological hypertrophy, becomes the major mechanism through which ventricular mass increases after a few months of post-natal life 3,4 ( Figs. 4-3 and 4-4 ). The stimulus is the normal developmental increase in mural stress and work. 12,13 This process is also present prenatally. Excessive pressure overload will induce hypertrophy, in addition to hyperplasia, in the fetal heart. 14

The developmental process that results in physiological hypertrophy is associated with changes in the shape and size of the cardiac myocytes ( Figs. 4-3 to 4-5 ). For example, the average length of the cardiac myocyte in the newborn rat is approximately 20 μm, while that of the 11-day-old rat is 45 μm. 4 Only an increase of one-fifth in cross sectional area accompanies this doubling of cell length. The immature cardiac myocyte changes from being relatively ovoid to the long tetrahedral shape of the adult myocyte (see Fig. 4-3 ). A further postnatal increase in cell length is seen in the adult heart, with cell lengths of 150 μm and longer being achieved in the mammal, and more than 300 μm in the bird. The cross sectional minor diameter increases from 10 to 20 μm following birth in the mammal 2 (see Fig. 4-4 ). These developmental changes are likely to have functional consequences, for example, the greater contribution of trans-sarcolemmal movement of calcium to the systolic [Ca 2+ ] i transient in the immature myocyte versus the greater contribution of calcium release from intracellular stores to be [Ca 2+] i transient in the adult myocyte ( Fig. 4-6 ). The greater contribution of trans-sarcolemmal calcium to the [Ca 2+ ] i transient in the immature myocyte may be reflected in the apparent great sensitivity of the infant heart following surgery to an increase in concentration of calcium in the plasma.


REGULATION OF CYTOSOLIC CALCIUM
The cascade underlying cardiac contraction is initiated by depolarisation of the transmembrane potential. Voltage-gated calcium channels are activated, extracellular calcium enters the myocyte, and calcium is released from intracellular stores into the cytosol. 15,16 The effects on sarcomeric function that follow from the systolic increase in cytosolic concentrations of [Ca 2+ ] i in the cytosol will be described in the section of the chapter devoted to the sarcomere. During diastole, [Ca 2+ ] i is about 100 nM, about 1/10,000 of extracellular calcium. The electrochemical gradient for entry of calcium is opposed primarily by the sarcolemmal Ca 2+ TPase, a system with high affinity but low capacity that regulates resting or diastolic [Ca 2+ ] i .
The L-type calcium channel, the dihydropyridine receptor, is the primary source for entry of calcium into the adult human cardiac myocyte. 17 With depolarisation, the L-type calcium channel is activated, and influx of calcium occurs ( Fig. 4-7 ). Following activation, and during the contraction, the channel closes as a consequence of an increase in [Ca 2+ ] i and further depolarisation, a feedback loop that limits the calcium current (I Ca ). Phosphorylation of the L-type calcium channel, secondary to β-adrenoreceptor stimulation, increases the calcium current. Another calcium channel, the T-type calcium channel, which is expressed in the embryonic heart, is activated at a more negative potential. 18,19

Exchange of calcium for sodium across the sarcolemma, through the Na + Ca 2+ exchanger, can occur in both directions 15,20 (see Fig. 4-7 ). This exchange of three sodium molecules for one calcium molecule is energy dependent. The direction of the exchange of sodium and calcium through the exchanger is based on its reversal potential. At more positive membrane potentials and higher [Na + ] i , influx is favoured through the exchanger. In some species, this exchanger may provide an amount of calcium to the systolic [Ca 2+ ] i transient similar to that provided by the L-type calcium channel. When the membrane potential is more negative, and [Ca 2+ ] i is higher, removal of calcium from the cell is favoured, helping restore [Ca 2+ ] i to diastolic levels. Operating in this mode, the exchanger demonstrates its role as a system with low affinity and high capacity, designed to deal with the intracellular loads of calcium.
The sarcoplasmic reticulum is the major intracellular site of release of calcium needed to support the [Ca 2+ ] i transient. 15 The reticulum contains specialised components, namely the junctional, corbular, and longitudinal components 2 ( Figs. 4-7 to 4-9 ). The junctional and corbular components contain calcium-binding calsequestrin, with 40 calcium molecules bound to each calsequestrin molecule, triadin, junction, and the ryanodine receptors, known as the RyRs, and representing the calcium-permeable ion channel of the reticulum. Corbular reticulum is not associated with the sarcolemma, while the junctional part is morphologically and functionally coupled to the transverse-tubules (see Fig. 4-8 ), forming dyads with the T-tubule sarcolemma, and peripheral couplings with the surface sarcolemma. The junctional and corbular components are located at the level of the Z-disc, where T-tubules are located. The T-tubular system, providing sarcolemmal extensions from the surface of the myocyte to deep within its core, is acquired with development, and is present in adult mammalian ventricular myocytes (see Figs. 4-7 and 4-8 ).

Entry of calcium into the cell triggers calcium-induced release from the sarcoplasmic reticulum (see Fig. 4-7 ). The calcium release units that support the systolic [Ca 2+ ] i transient are specialised junctional domains of the reticulum containing the L-type calcium channel, dihydropyridine receptors of the surface sarcolemma, and that of the T-tubule, the RyRs, triadin and junctin, and calsequestrin, the high capacity-low affinity calcium binding protein. 21 The latter four molecules form a supramolecular quaternary complex in the junctional and corbular parts of the sarcoplasmic reticulum. 16 Junctin binds calsequestrin within the calcium release units, but is not required for its localisation to the junctional and corbular components. 22 Junctin, also, regulates contractility but is not required for contraction. 23 The predominant ryanodine receptor in the heart is RyR2. The central role of trans-sarcolemmal influx of calcium in initiating calcium-induced release of calcium is evidenced by the effect of removing extracellular calcium. Activation of membranes in the absence of extracellular calcium does not result in excitation-contraction coupling. There is no [Ca 2+ ] i transient, and the myocyte fails to contract.
The close structural relationship between the junctional component of the sarcoplasmic reticulum and the L-type calcium channel, located in the sarcolemmal T-tubules, ensures that voltage-dependent opening of the L-type channel, and the associated flow of extracellular calcium into the cytosol, produces a very high [Ca 2+ ] i ryanodine receptor (see Fig. 4-7 ). In response to the increase in [Ca 2+ ] i with excitation, the ryanodine receptors are activated throughout the myocyte, and calcium flows into the cytosol from the sarcoplasmic reticulum, amplifying the effect of the L-type calcium channel current on the [Ca 2+ ] i transient. 24,25 The ryanodine receptors are, also, a scaffolding protein. The associated molecules in the release units, and associated kinases, phosphatases, and calmodulin are likely to modulate their function. 16,26,27
Cardiac relaxation follows the fall in the [Ca 2+ ] i transient to diastolic levels. Extrusion of calcium from the myocyte, and intracellular uptake of calcium, underlie this process. The major intracellular site of uptake is the longitudinal component of the sarcoplasmic reticulum (see Figs. 4-7 and 4-9 ). Although the mitochondria serve as a site for intracellular storage of calcium, they do not significantly contribute to the fall in the [Ca 2 + ] i transient. The longitudinal elements of the reticulum contain the sarcoplasmic version of calcium ATPase, known as SERCA, a transmembrane protein that translates calcium from the cytosol into the lumen of the sarcoplasmic reticulum. 15 Activity of SERCA is isoform dependent. 28 Of note, across development, cardiac myocytes express only SERCA2a. The longitudinal elements of the reticulum surround each sarcomere from Z-disc to Z-disc like a three-dimensional mesh, providing a system for rapid removal of the calcium bathed in the myofibrils (see Fig. 4-9 ). Calcium pumping is enhanced by [Ca 2+ ] i and decreased by [Ca 2+ ] within the sarcoplasmic reticulum. Activity of SERCA is inhibited by phospholamban. 29 Phosphorylation of phospholamban in response to β-adrenoreceptor stimulation removes its inhibitory effect on the activity of SERCA, and leads to a greater uptake of calcium into the longitudinal sarcoplasmic reticulum, providing a larger pool of calcium for release through the ryanodine receptors in subsequent contractions.

In summary, the systolic [Ca 2 + ] i transient is driven by modulation of the L-type calcium current, SERCA activity, the volume, organisation, and content of calcium in the sarcoplasmic reticulum, the number and properties of the ryanodine receptors, the sodium calcium exchanger, and the extent to which calsequestrin is saturated with calcium. Altogether, they modulate of the systolic [Ca 2 + ] i transient, provide the basis for the heart to vary its force of contraction from beat-to-beat, in other words the ability to modulate cardiac contractility. These processes underlie the increase in contractility that occurs with increase in heart rate, and those that follow from the introduction of an extrasystole. The extrasystole leads to greater content of calcium in the sarcoplasmic reticulum, allowing greater release of calcium, and a higher [Ca 2+ ] i transient in the post-extrasystolic contraction, and so post-extrasystolic potentiation ( Fig. 4-10 ).

DEVELOPMENT AND REGULATION OF CYTOSOLIC CALCIUM
The [Ca 2+ ] i transient increases postnatally in the mammalian ventricular myocyte 30 ( Fig. 4-11 ). In the immature heart, the [Ca 2+ ] i transient in response to excitation-contraction coupling has a greater dependence on trans-sarcolemmal influx of calcium 31 (see Fig. 4-6 ). Developmental changes in the sarcoplasmic reticulum, the calcium release units, constituted in its specialised junctional domains, 2,22 and expression of the sodium calcium exchanger, are all pertinent to this observation. 32,33

The organisation, differentiation, and relative volume of the sarcoplasmic reticulum increase with development, and the size and frequency of the specialised couplings with the surface and T-tubular sarcolemmal units that contain the elements for release of calcium increase with development. 21,22 The T-tubular system is acquired with development in the mammalian ventricular myocyte. The relative volume of the cells comprising the sarcoplasmic reticulum increases during late gestation, and following birth, 2,34 and the structure of the release units changes with development. In the immature myocyte, ryanodine receptors extend from the corbular component onto the surface of the longitudinal elements, while calsequestrin, located within the lumen of the corbular component in the adult, extends into the lumens of the longitudinal elements in the neonatal myocyte ( Fig. 4-12 ). The expression and targeting of calsequestrin to the junctional and corbular components is an early embryonic event that is followed by the expression of junctin and triadin. The developmental localisation of calsequestrin within the junctional and corbular components may be related to a marked post-natal increase in expression of junctin, and its binding of calsequestrin within the specialised region of the sarcoplasmic reticulum. 35 The fixed structural relation between the corbular components and the Z-disc are acquired post-natally (compare Figs. 4-9 and 4-12 ) in mammals that are not born ready to flee the nest as neonates. The lack of differentiation of the sarcoplasmic reticulum, and its ordered relation with other membranes in the immature myocyte, prevents the close coupling of the L-type calcium channel and the ryanodine receptors. This is likely, in the presence of a lower L-type calcium current density, to contribute to the slower rates of rise and lower peak systolic [Ca 2+ ] i transient in the immature myocyte 30 (see Fig. 4-11 ).

Function of the sarcoplasmic reticulum also changes with development as measured by increases in activity and efficiency of SERCA. These developmental changes in activity and efficiency will result in a more effective uptake of calcium by the sarcoplasmic reticulum, providing more calcium to be released during a subsequent contraction and, so, a developmental increase in the range over which the force of contraction can be modulated.
The developmental changes in the structural and biochemical properties of the sarcoplasmic reticulum are reflected in interventions that affect release of calcium by the ryanodine receptors. Ryanodine itself, which inhibits release of calcium by the receptors, does not affect the development of force by fetal myocardium, including that of humans. Within a few days following birth, however, ryanodine markedly attenuates the force of contraction. 36,37 Caffeine, which increases release of calcium by the receptors, has little effect on neonatal myocardium, while enhancing contractility in the adult. These findings support the importance of the developmental acquisition of the components underlying calcium-induced release of calcium, and the consequent decreased dependence of the adult heart on trans-sarcolemmal influx of calcium (see Fig. 4-6 ).
The greater role of contribution of the sarcoplasmic reticulum to the [Ca 2+ ] i transient in the adult heart is evidenced by post-extrasystolic potentiation and the restitution of contractility (see Figs. 4-10 and 4-13 ). Post-extrasystolic potentiation increases with maturation. 38 In addition, the restitution of contractility following a contraction (see Fig. 4-13 ) is also acquired with maturation. 2,38 Altogether, these findings demonstrate that the developmental increase in the amount, organisation, and structure of the sarcoplasmic reticulum and the calcium release units have a fundamental role in the developmental increase in the peak [Ca 2+ ] i transient in response to the L-type calcium current with activation.

There are two calcium channels expressed in the myocardium during development, the T-type and the L-type, the latter being dihydropyridine-sensitive. Early in development, a T-type calcium current is present, while in the adult heart, very little, if any, T-type calcium channel is expressed. 18 The developmental changes in the L-type calcium current density are more complex. In general, it increases with development (see Fig. 4-11 ). This increase may be related to an increase in the number of channels, or to differential expression of the α- and β-subunit isoforms. Importantly, the developmental increase in the L-type calcium current density provides a greater trigger for calcium-induced release of calcium.
The activity and expression of the sodium calcium exchanger increase during fetal life to a plateau, and subsequently fall rapidly during postnatal development. 33 The exchanger, working in a reverse mode, provides an important mechanism for influx of calcium to support the systolic [Ca 2+ ] i transient in the neonatal myocyte. 39 Working in the forward mode, the exchanger provides an effective mechanism for removal of calcium from the cytosol, bringing about relaxation. 15 Its effectiveness in removing calcium from the cell in association with a decreased uptake of calcium by the immature sarcoplasmic reticulum may result in little calcium being taken up by the immature sarcoplasmic reticulum, and so decreasing in the immature myocyte the calcium available for release from the sarcoplasmic reticulum in subsequent contractions.
Sodium potassium ATPase, a pump that regulates trans-sarcolemmal concentrations of the sodium and potassium ions, indirectly affects calcium within the cell. Cardioactive glycosides, for example digoxin, selectively bind to and inhibit the sodium potassium ATPase. Inhibition of this enzyme is thought to lead to elevation of sodium ions, with consequent increase in cellular and sarcoplasmic reticulate calcium via the exchanger. 40 Of note, other mechanisms for glycoside-induced enhancement of contractility have been suggested, including direct activation of ryanodine receptors, and increased selectivity by calcium of sodium channels. 41
Developmental changes have been found in the relative amount of the activity of the enzyme, and expression of its isoforms. Tissue-specific developmentally regulated and differentially localised expression of the α-subunit isoforms, the catalytic subunit, and differential effects of a β-subunit isoforms on affinity for sodium suggests that these isoforms have distinct roles. 42–44 These isoforms have different sensitivities and affinities for binding to cardiac glycosides. Myocardial contractility in the immature heart is positively affected by digoxin. 45,46 The differential expression of the isoforms, and the developmental regulation of this system, suggest that, in the human, α-subunit isoforms may contribute to maturational differences in the response to digoxin.
THE SARCOMERE
The sarcomere, the force-producing unit of the heart, is present at all stages of development. It is made up of a lattice-like highly organised arrangement of thick and thin filaments ( Fig. 4-14 A and B). The thick filament is a bipolar structure about 1.5 μm in length containing myosin heavy chain and multiple accessory proteins (see Fig. 4-14B ). Myosin is asymmetrically shaped, and is made up of two heavy chains and two pairs of associated light chains. 47 The heavy chains form two globular domains, the heads at one end of the molecule containing ATPase and a long rod-like coiled-coil domain. Molecules of the heavy chain are made up of homodimers or heterodimers of cardiac α- and β-units. The α-homodimers have the highest activity of ATPase, the α-β heterodimers have low immediate activity, and β-homodimers have the lowest activity. The long rod domains form the filament backbone of the thick filament, while the heads lie on the surface of the thick filament in a position that allows formation of strong cross-bridges with actin monomers in the adjacent thin filament. 48,49 Production of force, and sarcomeric shortening, require cyclic binding of the myosin heads to actin in the presence of calcium and adenosine triphosphate. Adenosine triphosphate is converted by the myosin ATPase into chemical energy, which is then converted into mechanical energy, moving the myosin head on actin, generating force and so sarcomeric shortening, and ejection of blood from the ventricle. Sarcomeric length affects the number of sites of actin available for binding with myosin. Based on the length of the thick filaments, and the central region of the thick filament that is bare of myosin heads, the optimum sarcomeric length for interaction between actin and myosin, and formation of cross-bridges, is from 2.0 to 2.2 μm ( Fig. 4-15 ). The length of the sarcomere also modulates the sensitivity of myofilamentous development of force to calcium through increasing affinity to troponin C, with an increase in sarcomeric length, as discussed below (see Fig. 4-18 ).


The accessory proteins in the thick filaments include an essential light chain, and a regulatory light chain, that are associated with each myosin head. 50,51 Phosphorylation of the regulatory light chain increases the sensitivity of myofilamentous development of force to calcium 52 (see Fig. 4-18 ). Other accessory proteins are myosin binding protein C, myomesin, m-protein, and titin 53–55 ( Fig. 4-16 ). The C binding protein modulates assembly of myosin, and its interaction with actin, stabilises the thick filaments, and interacts with actin. In response to β-adrenoreceptor stimulation, the single cardiac isoform of the C binding protein is phosphorylated by cyclic-AMP-dependent protein kinase A. 56 Titin, a long elastic protein, with a molecular weight of nearly 3000 kDa, keeps the thick filaments of the sarcomere centred between the Z-discs, maintains the integrity of the sarcomere, and supports, in part, its passive tension. 55 The titin filaments extend from the Z-discs, independent of the thin filaments, and attach to and continue along the thick filament, ultimately extending to and attaching to the next Z-disc (see Fig. 4-16 ).
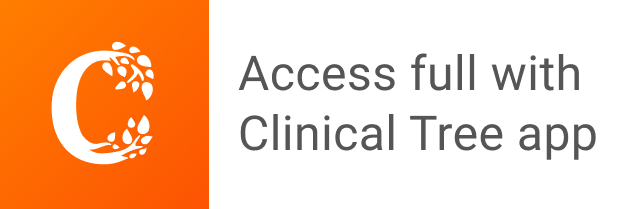