Attention ASE Members:
The ASE has gone green! Visit www.aseuniversity.org to earn free continuing medical education credit through an online activity related to this article. Certificates are available for immediate access upon successful completion of the activity. Nonmembers will need to join the ASE to access this great member benefit!
Preamble
Aortic pathologies are numerous, presenting manifestations are varied, and aortic diseases present to many clinical services, including primary physicians, emergency department physicians, cardiologists, cardiac surgeons, vascular surgeons, echocardiographers, radiologists, computed tomography (CT) and magnetic resonance (MR) imaging (MRI) imagers, and intensivists. Many aortic diseases manifest emergently and are potentially catastrophic unless suspected and detected promptly and accurately. Optimal management of these conditions depends on the reported findings from a handful of imaging modalities, including echocardiography, CT, MRI, and to a lesser extent invasive aortography.
In the past decade, there have been remarkable advances in noninvasive imaging of aortic diseases. This document is intended to provide a comprehensive review of the applications of these noninvasive imaging modalities to aortic disease. Emphasis is on the advantages and disadvantages of each modality when applied to each of the various aortic diseases. Presently, there is a lack of consensus on the relative role (comparative effectiveness) of these imaging modalities. An attempt has been made to determine first-line and second-line choices for some of these specific conditions. Importantly, we have emphasized the need for uniform terminology and measurement techniques. Whenever possible, these recommendations are evidence based, following a critical review of the literature. In some instances, the recommendations reflect a consensus of the expert writing group and include “vetting” by additional experts from the supporting imaging societies.
Because of the importance of prompt recognition to their successful treatment, this review emphasizes acute aortic syndromes (AAS), such as aortic dissection and its variants (e.g., intramural hematoma [IMH]), rupture of ascending aortic aneurysm, aortic trauma, and penetrating ulcer. Other entities, such as Takayasu aortitis (TA), giant-cell (temporal) arteritis (GCA), and mycotic aneurysm, are discussed briefly. Less common aortic diseases such as aortic tumors (because of their rarity) and congenital anomalies of the coronary arteries, aortic arch, and sinus of Valsalva aneurysms are not addressed. Several other topics are also beyond the scope of this review, including the important and emerging role of genetics in the evaluation and management of aortic diseases. Moreover, this document is not intended to replace or extend the recommendations of prior excellent guidelines in decision making and management for these conditions.
To summarize, the focus of this document is the fundamental role of the major noninvasive imaging techniques. In addition to clinical acumen and suspicion, knowledge of these imaging modalities is crucial for the assessment and management of the often life-threatening diseases of the aorta.
Preamble
Aortic pathologies are numerous, presenting manifestations are varied, and aortic diseases present to many clinical services, including primary physicians, emergency department physicians, cardiologists, cardiac surgeons, vascular surgeons, echocardiographers, radiologists, computed tomography (CT) and magnetic resonance (MR) imaging (MRI) imagers, and intensivists. Many aortic diseases manifest emergently and are potentially catastrophic unless suspected and detected promptly and accurately. Optimal management of these conditions depends on the reported findings from a handful of imaging modalities, including echocardiography, CT, MRI, and to a lesser extent invasive aortography.
In the past decade, there have been remarkable advances in noninvasive imaging of aortic diseases. This document is intended to provide a comprehensive review of the applications of these noninvasive imaging modalities to aortic disease. Emphasis is on the advantages and disadvantages of each modality when applied to each of the various aortic diseases. Presently, there is a lack of consensus on the relative role (comparative effectiveness) of these imaging modalities. An attempt has been made to determine first-line and second-line choices for some of these specific conditions. Importantly, we have emphasized the need for uniform terminology and measurement techniques. Whenever possible, these recommendations are evidence based, following a critical review of the literature. In some instances, the recommendations reflect a consensus of the expert writing group and include “vetting” by additional experts from the supporting imaging societies.
Because of the importance of prompt recognition to their successful treatment, this review emphasizes acute aortic syndromes (AAS), such as aortic dissection and its variants (e.g., intramural hematoma [IMH]), rupture of ascending aortic aneurysm, aortic trauma, and penetrating ulcer. Other entities, such as Takayasu aortitis (TA), giant-cell (temporal) arteritis (GCA), and mycotic aneurysm, are discussed briefly. Less common aortic diseases such as aortic tumors (because of their rarity) and congenital anomalies of the coronary arteries, aortic arch, and sinus of Valsalva aneurysms are not addressed. Several other topics are also beyond the scope of this review, including the important and emerging role of genetics in the evaluation and management of aortic diseases. Moreover, this document is not intended to replace or extend the recommendations of prior excellent guidelines in decision making and management for these conditions.
To summarize, the focus of this document is the fundamental role of the major noninvasive imaging techniques. In addition to clinical acumen and suspicion, knowledge of these imaging modalities is crucial for the assessment and management of the often life-threatening diseases of the aorta.
I
Anatomy and Physiology of the Aorta
A
The Normal Aorta and Reference Values
The aorta is the largest and strongest artery in the body; its wall consists of three layers: the thin inner layer or intima, a thick middle layer or media, and a rather thin outer layer, or adventitia. The endothelium-lined aortic intima is a thin, delicate layer and is easily traumatized. The media is composed of smooth muscle cells and multiple layers of elastic laminae that provide not only tensile strength but also distensibility and elasticity, properties vital to the aorta’s circulatory role. The adventitia contains mainly collagen as well as the vasa vasorum, which nourish the outer half of the aortic wall and a major part of the media.
The elastic properties of the aorta are important to its normal function. The elasticity of the wall allows the aorta to accept the pulsatile output of the left ventricle in systole and to modulate continued forward flow during diastole. With aging the medial elastic fibers undergo thinning and fragmentation. The ordinary concentric arrangement of the laminae is disturbed. These degenerative changes are accompanied by increases in collagen and ground substance. The loss of elasticity and compliance of the aortic wall contributes to the increase in pulse pressure commonly seen in the elderly and may be accompanied by progressive dilatation of the aorta.
A geometrically complex organ, the aorta begins at the bulb-shaped root (level 1 in Figure 1 ) and then courses through the chest and abdomen in a candy cane–shaped configuration, with a variable orientation to the long axis of the body, until it terminates in the iliac bifurcation. The aorta consists of five main anatomic segments: the aortic root, the tubular portion of the ascending aorta, the aortic arch, the descending thoracic aorta, and the abdominal aorta. The most proximal part of the ascending aorta, the aortic root (segment I in Figure 1 ), includes the aortic valve annulus, aortic valve cusps, coronary ostia, and sinuses of Valsalva. Distally the root joins the tubular portion of the ascending aorta (segment II) at an easily recognized landmark termed the sinotubular junction (STJ). The tubular portion of the ascending aorta extends from the STJ to the origin of the brachiocephalic artery. This relatively long segment is subdivided into segment IIa, which extends from the STJ to the pulmonary artery level, and segment IIb, from the pulmonary artery level to the brachiocephalic artery. The aortic arch (segment III) extends from the brachiocephalic artery to the left subclavian artery. The descending thoracic aorta (segment IV) may be subdivided into the proximal part (segment IVa), which extends from the left subclavian artery to the level of the pulmonary artery, and the distal part (segment IVb), which extends from the level of the pulmonary artery to the diaphragm. The abdominal aorta (segment V) may be subdivided into the proximal part (segment Va), which extends from the diaphragm to the ostia of the renal arteries, and the distal part (segment Vb), from the renal arteries to the iliac bifurcation.

1
Normal Aortic Dimensions
Because of the ease with which it can be visualized and its clinical relevance, the aortic root is the segment for which the greatest amount of data are available. Several large studies have reported normal aortic root diameters in the parasternal long-axis view by two-dimensional (2D) transthoracic echocardiography (TTE). Measurement of the aortic root diameter should be made perpendicular to the axis of the proximal aorta, recorded from several slightly differently oriented long-axis views. The standard measurement is taken as the largest diameter from the right coronary sinus of Valsalva to the posterior (usually noncoronary) sinus. Most studies report aortic root diameter measurements at end-diastole using the leading edge–to–leading edge technique ( Figure 2 ).

In adults, aortic dimensions are strongly positively correlated with age and body size. They are larger in men than in women of the same age and body size. Although in several reports, aortic diameters have been normalized to body surface area (BSA), this approach has not been entirely satisfactory because it is systematically lower in smaller than in larger normal adults. Fortunately, among children, the regression line of aortic diameter and height (rather than BSA) has a near-zero intercept, so that normalization to height has proved to be a simple and accurate alternative in growing children. Benchmark values from which the guidelines have been taken come from the work of Roman et al ., who reported normal root dimensions for three age groups ( Figure 3 ).

The upper limit of normal aortic diameter has been defined as 2 SDs greater than the mean predicted diameter. The Z score (the number of SDs above or below the predicted mean normal diameter) is a useful way to quantify aortic dilatation. Among normal subjects, 95.4% have Z scores between −2 and 2. Therefore, an aortic diameter can be considered dilated when the Z score is ≥2. Using the Z score allows comparison of a given patient’s aortic size at different time points, accounting for the effects of advancing age and increasing body size, thus distinguishing normal from pathologic growth. The Z score is therefore particularly useful for evaluating growing children.
It should be mentioned that aortic root dimensions may be increased by the hemodynamic effects of both endurance and strength exercise training in competitive athletes. This aortic root enlargement appears to be greater at the sinuses of Valsalva than at the aortic annulus or STJ. However, it should be emphasized that the effects of exercise training on aortic diameters are relatively small and that marked enlargement should suggest a pathologic process.
Recently, making use of a database consisting of a multiethnic population of 1,207 apparently normal adolescents and adults ≥15 years of age, investigators devised equations to predict mean normal aortic root diameter and its upper limit by age, body size (BSA or height), and gender ( Table 1 for men and Table 2 for women). These equations have been used graphically to depict the upper limits of the 95% confidence interval for normal aortic root diameter using surfaces to depict the interacting effects of age and body size (see Figure 4 for men and Figure 5 for women).
Age (y) | ||||||
---|---|---|---|---|---|---|
15–29 | 30–39 | 40–49 | 50–59 | 60–69 | ≥70 | |
Mean normal (cm) | 3.3 | 3.4 | 3.5 | 3.6 | 3.7 | 3.8 |
Upper limit of normal (cm) (95% CI) | 3.7 | 3.8 | 3.9 | 4.0 | 4.1 | 4.2 |
Age (y) | ||||||
---|---|---|---|---|---|---|
15–29 | 30–39 | 40–49 | 50–59 | 60–69 | ≥70 | |
Mean normal (cm) | 2.9 | 3.0 | 3.2 | 3.2 | 3.3 | 3.4 |
Upper limit of normal (cm) | 3.3 | 3.4 | 3.6 | 3.6 | 3.7 | 3.9 |

A noncontrast gated cardiac computed tomographic study, including 4,039 adult patients, showed age, BSA, gender, and hypertension to be directly associated with thoracic aortic diameters perpendicular to the long axis of the aorta. These associations are concordant with those from echocardiographic studies. In another recent large study using similar methodology, the mean value of the diameters of the ascending aorta was 1.8 ± 0.2 cm/m 2 and of the descending thoracic aorta was 1.4 ± 0.2 cm/m 2 , with the upper limits of normal being 2.1 and 1.8 cm/m 2 , respectively. However, more accurate normal values of thoracic aortic diameters may be obtained by anatomically correct double-oblique short-axis images using electrocardiographically gated multidetector CT or by MRI of axially oriented aortic segments. The upper limits of normal are 3.7 cm for the aortic root at the sinuses, 3.6 cm for the ascending aorta and 2.5 cm for the descending thoracic aorta by CT, and 2.5 cm for the descending thoracic aorta and 2.0 cm for the upper abdominal aorta by MRI. As with echocardiography, aortic root and ascending aortic diameters increase significantly with age and BSA on CT and MRI. Aortic root diameters increase 0.9 mm per decade in men and 0.7 mm per decade in women.
The establishment of normative values and reference ranges, taking into account aging and gender, is of great importance for diagnosis, prognosis, serial monitoring, and determining the optimal timing for surgical intervention. Normal values and proximal aortic diameters have been reported using different imaging techniques, from the pioneer studies based on M-mode and 2D echocardiography to more recent studies obtained using CT and MRI. Despite differences in image acquisition methods, temporal and spatial resolution, and signal-to-noise ratios, CT, MRI, TTE, and transesophageal echocardiography (TEE) have evolved as near equal standards for assessing aortic root size. Each of these modalities has advantages and disadvantages, which have been discussed. It should be emphasized that normal aortic diameters vary systematically by age, gender, and body size, and reference values indexed to those parameters have been provided. Last, it is critically important to emphasize not only methodologic variance but also inter- and intraobserver variability. In several studies, variability of measurement of proximal aortic diameters ranges from 1.6 to 5 mm. Given this degree of variability, apparent small changes in proximal aortic diameters on serial computed tomographic examinations may be within the range of measurement error. Accordingly, for all imaging techniques, we recommend that changes of ≤3 mm by electrocardiographically gated CT and ≤5 mm without electrocardiographic gating be viewed with caution and skepticism.
B
How to Measure the Aorta
Accurate and reproducible measurements of aortic dimensions are necessary for the detection and classification of aortic disease and for guiding therapeutic decisions. Modern imaging modalities enable one to make measurements far more accurately than did invasive contrast angiography, the only tool originally available.
Echocardiography, CT, and MRI each has particular strengths and limitations but can be adapted for the acquisition of views that allow measurement of the diameter or cross-sectional area of different segments of the aorta ( Figure 1 ).
1
Interface, Definitions, and Timing of Aortic Measurements
The American Society of Echocardiography (ASE) proposed standards for measurement of the aortic root in 1978. The ASE recommended measurement at end-diastole from the leading edge of the anterior root wall to the leading edge of the posterior aortic root wall. This technique was believed to minimize the impact of “blooming” of bright reflectors on this measurement. The ASE-recommended method was followed in many important clinical and epidemiologic studies that have reported normal limits for individuals of differing body size and age, and these normal limits have been incorporated into multiple guidelines for imaging in adults ( Figures 4 and 5 ). As a consequence, much of the available data on normal aortic root size as well as on the prevalence and prognostic significance of aortic dilatation in adults have emerged from echocardiography.
Societal guidelines for measurement by CT or MRI are not currently available. Consequently, uniformity in measurement methods is lacking. Many research and clinical studies using these modalities have reported aortic measurements made from inner edge to inner edge on electrocardiographically gated or nongated images. The 2010 guidelines for the diagnosis and management of thoracic aortic disease took the opposite approach, recommending measurement of aortic diameter between external surfaces to avoid confounding by intraaortic thrombus or atheroma, as is commonly found in the abdominal but not in the ascending aorta. Furthermore, there is no standardized “trigger time” (end-systole vs end-diastole) for image acquisition. Thus, the use of multiple imaging modalities such as CT, MRI, and 2D and three-dimensional (3D) echocardiography has led to nonuniformity in measurement techniques. Moreover, there is currently no standardized approach for reconciling aortic measurements across imaging modalities (echocardiography, CT, MRI, aortography) by trigger time (end-systole vs end-diastole) or by edge selection (leading edge, inner-inner, outer-outer). This writing committee had hoped to recommend a uniform and consistent measurement technique to minimize differences among these various imaging modalities. However, after much consideration, the group recommends that echocardiographic measurements continue to be made in the standard fashion from leading edge to leading edge, at end-diastole, and perpendicular to the long axis of the aorta. The advantages of end-diastolic measurements include greater reproducibility (because aortic pressure is most stable in late diastole) and the ease of identification of end-diastole by the onset of the QRS complex. Although other techniques use the inner edge–to–inner edge approach, there are currently insufficient data to warrant a change for echocardiography. Available data suggest that the echocardiographic leading edge–to–leading edge approach produces values comparable with those produced by the inner edge–to–inner edge approach on CT and MRI, is reproducible, and links to a large body of historical and prognostic data that have long guided clinical decision making.
For all modalities, it is desirable, whenever possible, to specify the locations of measurements, by referencing them to a given landmark. For example, with TEE, a measurement of the maximal diameter of the ascending aorta may be reported by its distance from the STJ. In the descending thoracic aorta, reference to the location of a measurement or abnormality is usually made by its distance from the incisors. Similar attempts should be made for measurements and findings with CT and MRI.
2
Geometry of Different Aortic Segments: Impact on Measurements
Accurate and reproducible measurement of aortic diameter or cross-sectional area in a given segment requires three measurements of its diameter perpendicular to the long axis. In most cases, the largest correctly oriented measurement is reported.
a
Aortic Annulus
Although the aortic annulus is approximately circular in children and young adults, it may become elliptical in older adults. Thus, 3D imaging by CT or echocardiography or 2D imaging in multiple planes (e.g., long-axis or sagittal and coronal planes) is required to measure a diameter that is accurate enough to be used when selecting patients for transcatheter aortic valve replacement ( Figure 6 ).
b
Sinuses of Valsalva and STJ
Aortic root diameter can be measured perpendicular to its long axis by 2D echocardiography or in analogous nontrue coronal and sagittal plane by MRI or CT. The variability in this measurement resulting from the orientation of the aortic root is overcome by choosing the largest diameter measured from the right coronary sinus of Valsalva to the posterior (usually noncoronary) sinus, parallel to the aortic annulus and perpendicular to the long axis of the proximal aorta in several slightly differently oriented long-axis views. Failure to search for the largest correctly oriented measurement can lead to underestimation of aortic root diameter. Aortic root diameter is commonly measured by CT or MRI between the inner edges from commissure to opposite sinus ( Figure 7 ). Diameters measured using the sinus-to-sinus method are generally a mean of 2 mm larger than those measured by the sinus-to-commissure method ( Figure 8 ). However, using the sinus-to-sinus method has several advantages, including the ease of detecting cusp margins in computed tomographic or MRI transverse planes, close agreement with echocardiographic measurements, and greater feasibility in bicuspid valves. Thus, for aortic measurements by CT and MRI, it is recommended to average the three sinus-to-sinus measurements in end-diastole in the sinus-of-Valsalva plane. When the sinuses are unusually asymmetric, it may be preferable to report the three measurements individually.
c
Ascending Aorta and More Distal Segments
The same basic principles apply to obtaining correct measurements of the other aortic segments. Conventional imaging by all modalities and techniques can be used to measure the diameter of aortic segments that are oriented along the long axis of the body. However, the necessity to avoid oblique imaging that can overestimate the aortic diameter applies to the aortic arch and to portions of the descending thoracic and abdominal aorta that may take a tortuous course ( Figure 9 ).
We emphasize that there is no standardized method for measuring the aorta across imaging modalities (echocardiography, CT, MRI, aortography). Although one of the major goals of this writing committee was to provide a uniform and universally accepted method to minimize differences among these various imaging modalities, no consensus could be reached. After much consideration, it is recommended that echocardiographic measurements continue to be made from leading edge to leading edge. Although other techniques use inner edge–to–inner edge or outer edge–to–outer edge approaches, there are currently insufficient data to warrant a change for echocardiography. Available data suggest that the echocardiographic leading edge–to–leading edge approach gives larger measurements compared with the inner edge–to–inner edge approach on CT (average difference, 2 mm), and the leading edge–to–leading edge method links to a large body of historical and, more important, prognostic data that influence decision making. Out of concern that patient management might be adversely affected (i.e., intervention might be delayed, leading to a catastrophic complication such as rupture or dissection) by switching to a new protocol that would lead to a smaller measurement, it was decided to continue to recommend the leading edge–to–leading edge approach.
C
Aortic Physiology and Function
The aorta functions as both a conduit and a reservoir. Its elastic properties allow it to expand in systole and recoil during diastole. Thus, under normal conditions, a large proportion (up to 50%) of the left ventricular stroke volume is stored in the aorta (mainly in the ascending aorta) at end-systole, and the stored blood is then propelled forward during diastole into the peripheral circulation. This reservoir function is important for maintaining blood flow and arterial pressure throughout the cardiac cycle. The thoracic aorta is more distensible than the abdominal aorta because its media contains more elastin. Aortic distensibility declines with age and as a result of premature degeneration in elastin and collagen associated with some disease states. During left ventricular systole, this loss of aortic wall compliance results in increased systolic pressure and pulse pressure and, in turn, aortic dilatation and lengthening. The compliance of the aortic wall may be estimated by assessing change in aortic volume in relation to the simultaneous change in aortic pressure. This may be assessed locally by diameter or area change through the cardiac cycle in relation to pressure change (e.g., distensibility) or regionally by determination of the velocity of the pulse wave.
1
Local Indices of Aortic Function
Techniques that provide accurate definition of the aortic diameter or volume in systole and diastole can be used to evaluate the elastic properties of the aorta. The most commonly applied indices for clinical purposes are aortic distensibility and the stiffness index, which is less dependent on blood pressure. Aortic distensibility and the stiffness index can be determined from the changes in the aortic diameter from systole to diastole and from changes in the arterial pressure using the following formula:
Distensibility ( 10 − 3 · mm Hg − 1 ) = Area systole − Area diastole Area diastole · Pulse pressure · 1,000.
For these calculations, the pulse pressure should be measured ideally at the same level of the aorta at which the aortic diameter is measured. In clinical practice, however, brachial artery pressure can be used, even though the pulse pressure obtained from the brachial artery may be slightly higher than that obtained from the aorta because of the amplification phenomenon, which is more apparent in young individuals.
2
Regional Indices of Aortic Stiffness: Pulsewave Velocity (PWV)
PWV is defined as the longitudinal speed of the pulsewave in the aorta. PWV is inversely related to aortic elasticity. Hence, a stiffer aorta will conduct the pulsewave faster than a more compliant aorta. Central pressure, at the level of the ascending aorta, is produced as a combination of the antegrade wave from the left ventricle and the retrograde “reflective” waves from the periphery. In young individuals, because the aorta is more elastic, the pulsewave speed is low, so the retrograde flow arrives in the proximal aorta during diastole. As a result of aortic stiffening, the PWV increases, and the retrograde flow arrives in the proximal aorta earlier in systole, leading to a greater LV afterload and decreased diastolic pressure.
Reported normal values for invasively determined PWV measurements in middle-aged humans are 4.4 ± 0.4 m/sec in the aortic root, 5.3 ± 0.2 m/sec in the proximal descending thoracic aorta, 5.7 ± 0.4 m/sec in the distal thoracic descending aorta, 5.7 ± 0.4 m/sec in the suprarenal abdominal aorta, and 9.2 ± 0.5 m/sec in the infrarenal aorta.
Carotid-femoral PWV is considered to be the gold-standard measure of arterial stiffness, especially because it is simple to obtain and because multiple epidemiologic studies have demonstrated its predictive value for cardiovascular events. However, the ability of a given individual’s PWV value to predict aortic events has not been previously evaluated. A recent expert consensus adjusted this threshold value to 10 m/sec by using the direct carotid-to-femoral distance. The main limitation of PWV interpretation is that it is significantly influenced by arterial blood pressure. Multimodality imaging techniques provide a unique opportunity to assess aortic PWV by the formula:
Aortic PWV ( m / sec ) = Distance ( m m ) Transit t i m e ( m sec )
Echocardiography can accurately estimate the transit time between aortic levels by the subtraction of the time between a fixed reference in the QRS complex and the beginning of the flow at the two levels studied. Distance can be grossly estimated externally with a tape.
MRI can measure the PWV using the transit time of the flow curves from a phase-contrast acquisition. Transit time can be calculated by the upslope approach, which has been described previously and correlates more with age and aortic stiffness indices than point-to-point approaches such as foot-to-foot and half-maximum methods. Distance can accurately be measured at the centerline of the aorta between aortic levels studied.
A normal-size aorta may be functionally abnormal. Thus, determination of aortic function may help define the nature of the underlying disease and give prognostic information in some diseases. This was emphasized by Vriz et al ., who stated that aortic stiffness should be taken into account when increases in aortic diameter are detected.
In summary, aortic biophysical properties can be easily and reliably assessed by imaging techniques, particularly Doppler echocardiography and phase-contrast MRI. This evaluation may provide important pathophysiologic and prognostic information that may have clinical implications both in disease states and in the general population.
II
Imaging Techniques
A
Chest X-Ray (CXR)
Most articles describing the use of imaging to evaluate patients with suspected AAS have focused on the role of CT, MRI, echocardiography, and aortography. Although routine CXR rarely provides a definitive diagnosis, it can provide several important diagnostic clues to aortic diseases that prompt further evaluation. Table 3 lists some of the common and uncommon plain CXR findings of aortic diseases.
|
Moreover, in cases of asymptomatic or chronic aortic diseases, CXRs may actually provide the first clue to aortic pathology. Importantly, CXR can identify other chest disorders that may contribute to a patient’s illness (e.g., pneumonia, pneumothorax, rib fracture). Nevertheless, although CXR may be valuable, it is neither sensitive nor specific for AAS. Moreover, normal results on CXR with respect to the aorta should never prevent or delay the further diagnostic evaluation of a patient with a suspected AAS.
In summary, although useful in drawing attention to the possibility of aortic disease, its low sensitivity, specificity, and interobserver agreement limit the role of the CXR.
B
TTE
The thoracic aorta should be routinely evaluated by TTE, which provides good images of the aortic root, adequate images of the ascending aorta and aortic arch in most patients, adequate images of the descending thoracic aorta in some patients, and good images of the proximal abdominal aorta. New advances in imaging quality and harmonic imaging have significantly improved the assessment of the aorta by TTE.
The aortic root and proximal ascending aorta are best imaged in the left parasternal long-axis view. The left lung and sternum often limit imaging of the more distal portion of the ascending aorta from this transducer position. In some patients, especially those with aortic dilatation, the right parasternal long-axis view can provide supplemental information. The ascending aorta may also be visualized in the apical long-axis (apical three-chamber) and apical five-chamber views and (especially in children) in modified subcostal views.
There is usually no clear echocardiographic delineation between the sinus and tubular portion of the ascending aorta, but occasionally a fibrotic or sclerotic ridge, located at the STJ, is imaged. This ridge may be prominent and should not be confused with vegetation, abscess, mass, atherosclerotic plaque, dissection flap, or supraaortic stenosis. The maximum diameter of the aorta is normally in the root (sinus portion), which is immediately distal to the aortic valve.
Echocardiographic measurements of the aortic root will vary in an individual patient at different levels ( Figure 10 ). The aortic diameter is smallest at the annulus and largest at the mid–sinuses of Valsalva. The tubular portion of the ascending aorta is typically about 10% smaller than the diameter at the sinus level. The aortic arch is usually easily visualized from the suprasternal view. Portions of the ascending and descending aorta can be visualized simultaneously. One or more of the three arch branches can usually be imaged: the left carotid and left subclavian arteries are identified in >90% of cases and the brachiocephalic (inniminate) artery in up to 90% ( Figure 11 ). Just distal to the left subclavian artery is the level of the ligamentum arteriosum, which is a common site of atherosclerosis, and a shelf or indentation (a ductus diverticulum) is sometimes imaged in this region.
The descending thoracic aorta is often incompletely imaged by TTE. A cross-sectional view of the descending thoracic aorta may be seen in the parasternal long-axis view, as it passes posteriorly to the left atrium near the atrioventricular groove. It can also be seen in short axis in the apical four-chamber view. By rotating the transducer 90°, a long-axis view of the midportion of the descending thoracic aorta may be obtained. A portion of the descending thoracic aorta can also be imaged from a suprasternal view. In patients with left pleural effusion, scanning from the back may also provide satisfactory views of the descending thoracic aorta. However, the distal descending thoracic aorta frequently cannot be imaged clearly because of reduced resolution in the far field. Moreover, physical characteristics of some patients exceed the limit of ultrasound penetration.
The normal descending thoracic aorta is smaller than both the aortic root and ascending aorta. As it descends, its diameter progressively narrows from 2.5 to 2.0 cm. Larger dimensions are reported in patients with hypertension, aortic valve disease, and coronary atherosclerosis. The aorta is consistently about 2 mm smaller in female than it is in male subjects.
A substantial portion of the upper abdominal aorta can be easily imaged in subcostal views, to the left of the inferior vena cava. This should be routinely performed as a part of a 2D echocardiographic study. Often the proximal celiac axis and the superior mesenteric artery can also be imaged. When present, aneurysmal dilatation, external compression, intra-aortic thrombi, protruding atheromas, and dissection flaps can be imaged, and flow patterns in the abdominal aorta can be assessed. The infrarenal abdominal aorta is best imaged as part of an abdominal ultrasound examination by use of a linear array probe.
In summary, to reliably evaluate patients with suspected aortic disease, the entire thoracic aorta must be imaged well. This is possible in some, but not all, patients on systematic TTE. TTE is particularly useful for evaluating the aortic root, and the ascending aorta and arch may also be adequately visualized in patients with good acoustic windows. TTE is less helpful for evaluating the descending thoracic aorta. However, TTE is an excellent screening tool for detecting aneurysms of the upper abdominal aorta.
C
TEE
TEE, introduced clinically in the late 1980s, has had a major impact on the evaluation of numerous diseases involving the aorta. TEE has two main advantages over TTE. First, superior image quality can be obtained from the use of higher frequency transducers than are possible with TTE. Second, because of the close proximity of the esophagus to the thoracic aorta, TEE provides high-quality imaging of nearly all of the ascending and descending thoracic aorta. TEE incorporates all the functionality of TTE, including 3D imaging, which can reliably interrogate cardiovascular anatomy, function, hemodynamics, and blood flow. The current multiplane TEE transducer consists of a single array of crystals that can be rotated electronically or mechanically around the long axis of the ultrasound beam in an arc of 180°. With rotation of the transducer array, multiplane TEE produces a continuum of transverse and longitudinal image planes.
1
Imaging of the Aorta
As mentioned, the anatomic proximity of the thoracic aorta and the esophagus allows superb visualization of the aorta using TEE. The multiplane transesophageal echocardiographic examination of the aorta is conducted as follows : with the tip of the transesophageal echocardiographic probe in the esophagus, the ascending aorta is best visualized from a 100° to 140° view: the image is analogous to the transthoracic echocardiographic parasternal long-axis view (but “flipped” upside down if the transesophageal echocardiographic probe “bang” is at the top). This view can be optimized by carefully rotating the transducer between 100° and 140°. Short-axis views of the aortic root and ascending aorta can be obtained from the 45° to 60° angle, usually with an anteflexed probe. From the midesophagus at 0°, the probe needs to be rotated posteriorly to obtain short-axis images of the descending thoracic aorta. While keeping the thoracic aorta in view, the probe can be withdrawn to image upper thoracic levels of the descending aorta or advanced to sequentially image the lower thoracic and upper abdominal aorta. With the transducer array at 90°, a longitudinal view of the aorta can be obtained.
By advancing the probe into the stomach, the proximal portion of the abdominal aorta and the celiac trunk can be seen. The mid and distal abdominal aorta are usually not seen because of difficulty maintaining good contact with the mucosa of the stomach. To obtain images of the arch, the transesophageal echocardiographic probe needs to be facing posteriorly and withdrawn from the midesophagus. With the transducer array at 90°, a short-axis view of the transverse arch can be obtained. It is usually possible to visualize the takeoff of the left subclavian artery, but the left common carotid and brachiocephalic arteries can be difficult or impossible to image and usually require careful clockwise rotation of the probe. A portion of the distal ascending aorta and proximal aortic arch may not be visible because of interposition of the trachea. This “blind spot” can be partially resolved with longitudinal views. An additional view, the deep transgastric view, can sometimes image the entire ascending aorta and often the proximal arch ( Figure 12 ).
D
Three-Dimensional Echocardiography
Real-time 3D TEE, a relatively new technology, appears to offer some advantages over 2D TEE in a growing number of clinical applications. However, as of this writing, there is limited information regarding the clinical application of this novel technology to the thoracic aorta.
Moreover, 3D TEE has some limitations. Like 2D TEE, it often fails to adequately visualize the distal ascending aorta and the aortic arch and its branches, because of interposition of the trachea. In addition, spatial imaging of the thoracic aorta is limited because of the 90° image sector, which is too narrow to include long segments of the thoracic aorta and therefore limits topographic orientation. In summary, recent advances in 3D TEE provide an opportunity to reconsider the role of TEE for diagnosing and monitoring patients with aortic diseases. Future experience will be required to verify its benefits and establish its value relative to CT and MRI.
E
Intravascular Ultrasound (IVUS)
IVUS is performed by introducing a miniature, high-frequency (10–30 MHz) ultrasound transducer mounted on the tip of a disposable catheter, through a large arterial (usually femoral) sheath, and advanced over conventional guidewires using fluoroscopic guidance. Less commonly, the IVUS imaging catheter can be inserted into the femoral vein, navigated into the inferior vena cava, and aimed at the adjacent aorta. IVUS produces an axial view that is a 360° real-time image. Consecutive axial images can be obtained during a “pullback” of the ultrasound catheter. This procedure can be safely performed in a few minutes.
Because of its intraluminal position, IVUS permits visualization of the aortic wall from the inside. This intraluminal perspective can provide information that supplements the other imaging modalities. Using the pullback technique, luminal diameter, cross-sectional area, and wall thickness can be measured. In addition to providing measurements, IVUS also provides qualitative information on nearly all aortic pathologies, including aortic aneurysms, aortic dissections, atherosclerosis, penetrating ulcers, and traumatic lesions ( Figures 13 and 14 ). Unlike TEE, IVUS can also determine the dissection characteristics in the abdominal aorta.
1
Limitations
The normal aorta appears on IVUS as a circular cross-sectional image with an intact wall and a clear lumen. The ultrasound catheter and the guidewire are seen within the lumen. In some instances, it can be difficult to obtain complete cross-sectional images of the aorta within a single frame of the image display at the arch and locations where the aorta is significantly dilated, because of difficulty maintaining the ultrasound catheter in a central and coaxial orientation and because of the limited penetration with high-frequency transducers. This limitation can be partially overcome by periodic reorientation of the ultrasound catheters. There are also concerns with IVUS measurements. Off-center measurements or those taken in tortuous portions of the aorta (tangential measurements on a curve) do not reflect a true centerline diameter, may provide an oblique slice, and are less accurate than centerline computed tomographic measurements. Another major limitation of IVUS is that it lacks Doppler capabilities (color Doppler can detect flow into small arteries, false luminal flow, and endoleaks). Last, the high cost of the disposable transducers and invasive nature of the technique limit IVUS for most clinical applications other than guidance of endovascular procedures.
F
CT
Multidetector computed tomographic scanners (≥64 detector rows) are the currently preferred technology for aortic imaging. Computed tomographic aortography (CTA) remains one of the most frequently used imaging techniques for diagnosis and follow-up of aortic conditions in acute as well as chronic presentations. This popularity reflects its widespread availability, accuracy, and applicability, even for critically ill patients or those with relative contraindications to MRI such as permanent pacemakers and defibrillators. Multidetector CT (MDCT) provides extensive z-axis coverage (in the long axis of the body), with high spatial resolution images acquired at modest radiation exposure within a scan time lasting a few seconds. Furthermore, CTA allows simultaneous imaging of vascular structures, including the vessel wall and of solid viscera. The minimization of operator variability and the capacity of delayed reprocessing of source images make it an ideal technique for comparative follow-up studies.
The latest innovations in clinical practice include electrocardiographically gated aortic computed tomographic studies leading to high-quality, precise imaging of the ascending aorta, as well as simultaneous evaluation of the coronary arteries ( Figure 15 ). Electrocardiographically gated CTA adds valuable information in the study of aortic pathology involving the aortic root and valve, in congenital heart disease, for simultaneous aortocoronary evaluation, for planning of endovascular therapy, for imaging of the postsurgical ascending aorta, and to show dynamic changes of true luminal compression in aortic dissection.
The main drawbacks of CT are the use of ionizing radiation and iodinated contrast media (ICM). Using optimal acquisition methods, large reductions in ionizing radiation dose can be achieved. These include the use of tube current modulation, prospective electrocardiographically triggered acquisitions, or tube voltage reductions to 80 to 100 kV. Radiation dose becomes most relevant in younger men and premenopausal women. Contrast-associated nephropathy may be avoided or significantly decreased by proper patient hydration and use of the minimum volume of low- or iso-osmolar ICM. The rate of adverse reactions to low-osmolar ICM in CT is approximately 0.15%, with most cases self-resolving and mild. Among patients with renal insufficiency, the rates of contrast-associated nephropathy are low. Pooled data from recently published prospective studies have shown an overall rate of contrast-associated nephropathy of 5% after intravenous injection of ICM in 1,075 patients with renal insufficiency, with no serious adverse outcomes (dialysis or death).
The current generation of computed tomographic scanners is able to significantly decrease the effective radiation dose and the total volume of ICM required for aortic imaging. Additionally, CT for follow-up of aortic expansion may be performed without ICM, relying on noncontrast images only.
1
Methodology
a
CTA
The combination of wide multidetector arrays with short gantry rotation times in ≥64-detector computed tomographic scanners results in standard acquisition times of 3 to 4 sec for the thoracic aorta and <10 sec for the thoracoabdominal aorta and the iliofemoral arteries.
The minimal technical characteristics of state-of-the-art CTA are a slice thickness of ≤1 mm and homogeneous contrast enhancement in the aortic lumen. Complete examination of the aorta from the supra-aortic vessels to the femoral arteries is needed for evaluation before transthoracic endovascular aortic repair (TEVAR), but as a general rule the scan length (anatomic scan range) on CTA should be individually tailored to avoid unnecessary exposure to ionizing radiation.
i
Noncontrast CT before Aortography
In the acute setting of a suspected AAS, it is important to initiate the protocol by a noncontrast thoracic computed tomographic scan to rule out IMH. This scan identifies concentrated hemoglobin in recently extravasated blood within the aortic wall that shows a characteristically high computed tomographic density (40–70 Hounsfield units), facilitates the characterization of the hematoma, can identify vascular calcifications, and provides a baseline examination for postcontrast evaluation.
ii
Electrocardiographically Gated CTA
Motion artifacts involving the thoracic aorta are evident in most (92%) standard nongated computed tomographic angiograms. Because of the limited temporal resolution of CT, imaging artifacts arising from the peduncular motion of the heart, the circular distension of the pulsewave, aortic distensibility, and the hemodynamic state may appear as a “double aortic wall” on standard nongated CTA. This finding may also lead to a false-positive diagnosis of a dissection flap and impair accurate measurement of the aortic root and ascending aorta.
Prospective or retrospective synchronization of data acquisition with the electrocardiographic tracing eliminates these artifacts, thereby improving the accuracy of diagnosis and reproducibility of aortic size measurements. Low-dose prospective electrocardiographically gated CT protocols have the advantage of decreased radiation exposure compared with the standard technique.
iii
Thoracoabdominal CT after Aortography
A late thoracoabdominal scan (∼50 msec after bolus injection) improves the detection of visceral malperfusion in the acute setting of aortic dissection, detects slow endograft leaks, distinguishes slow flow from thrombus in the false lumen, and allows alternative abdominal diagnoses in the absence of acute aortic pathology.
iv
Exposure to Ionizing Radiation
Radiation minimization protocols include limiting scan range, prospectively electrocardiographically triggered acquisitions, and using low tube voltage (80–100 kV) for low–body weight patients (<85 kg) without risking loss of diagnostic quality. Application of iterative reconstruction algorithms provide the opportunity for even larger reductions in scan acquisition parameters. Despite progress in radiation reduction, the use of alternative methods such as MRI and echocardiography remains a consideration for serial studies.
v
Measurements
In contrast with other aortic imaging techniques, CTA depicts the aortic wall, thereby permitting measurement of both the inner-inner (luminal) and outer-outer (total) aortic diameters. Imaging artifacts from the highly contrasted lumen frequently impair the visualization of a thin and healthy wall in the ascending aorta.
Multiplanar reconstruction of the axial source data can create aortic images in a plane perpendicular to the aortic lumen direction (double-oblique or true short-axis images of the aorta; see Figures 16 and 17 ). This method corrects shape distortions introduced by aortic tortuosity. In cases of noncircular aortic shape, both major and minor diameters should be measured. The manual procedure of double-oblique images is time consuming and may add observer variability. Automated aortic segmentation software is available at many institutions but, like most automated software, has limitations and requires manual adjustment.
The measurement technique must be highly reproducible to correctly assess follow-up studies. Accurate assessment of aortic morphologic changes can be achieved by side-by-side comparison of source axial images from two or more serial computed tomographic aortographic examinations with anatomic landmark synchronization and a slice thickness ≤1 mm. Electrocardiographically gated or triggered imaging is an additional refinement that further reduces variability, with a maximum interobserver variability of ±1.2 mm in the ascending aorta. Measures in the axial plane are valid only for aortic segments with a circular shape and craniocaudal axis, like the midascending and the descending aorta. Distortion in the axial image introduced by aortic tortuosity may be minimized by measuring the lesser diameter. Interobserver variability is always higher than intraobserver variability, suggesting that follow-up of aortic disease in a specific patient should be performed by a single experienced observer.
In summary, CTA is one of the most used techniques in the assessment of aortic diseases. Advantages of CTA over other imaging modalities include the short time required for image acquisition and processing, the ability to obtain a complete 3D data set of the entire aorta, and its availability. Moreover, MDCT permits a correct evaluation of the coronary arteries and aortic branch disease. Its main drawbacks are the radiation exposure and need for contrast administration.
G
MRI
MRI is a versatile tool for assessing the aorta and aorta-related pathologies. This imaging modality can be used to define the location and extent of aneurysms, aortic wall ulceration, and dissections and to demonstrate areas of wall thickening related to aortitis or IMH. MRI can also be used for preoperative and postoperative evaluation of the aorta and adjacent structures. Additionally, MRI can provide functional data, including quantification of forward and reverse aortic flow, assessment of aortic wall stiffness and compliance, and aortic leaflet morphology and motion ( Figure 18 ). All of this information is obtainable without the burden of ionizing radiation and, in some instances, without the need for intravenous contrast.
MR images are based on the signal collected from hydrogen nuclei, which align and process along the axis of the magnetic field when a patient enters the scanner. This precession can be altered by applying magnetic field pulses in a controlled fashion to create “pulse sequences.” After these pulse sequences are applied, the signal from the hydrogen nuclei is measured and then processed to produce MR images. The versatility of MRI can be attributed to the multiple types of pulse sequences that can be used to define structure, characterize tissue, and quantify function.
1
Black-Blood Sequences
Black-blood MRI sequences, acquired with spin-echo techniques, and often including inversion recovery pulses, are useful for defining morphology across a spectrum of aortic conditions without the need for intravascular contrast medium. With these sequences, the use of multiple radiofrequency pulses nulls the signal from moving blood, causing the dark blood appearance; mobile protons in stable or slowly moving structures (e.g., aortic wall) provide the signal in the image. Aortic wall morphology can be defined and tissue characterized with T1- and T2-weighted sequences and their variants, including T2-weighted dark-blood techniques and T2 turbo spin-echo and short-tau inversion recovery sequences ( Figure 19 ). Each of these imaging protocols has relative strengths and limitations; for example, T2-weighted MRI is sensitive to areas of increased water content, as is often noted in pathologic conditions, but is limited by relatively low signal-to-noise ratio. MRI of the thoracic aorta can be obtained with high spatial resolution, with in-plane resolution typically in the range of 1.5 × 1.5 mm and submillimeter acquisition achievable with more specialized MRI sequences.
2
Cine MRI Sequences
Bright-blood imaging with approaches such as steady-state free precession and gradient-echo techniques is useful for obtaining high–temporal resolution cine images of flow in the aorta. In these images, the blood pool is bright compared with the adjacent aortic wall, which is typically intermediate in signal. Cine imaging can demonstrate flow within aortic lumens (true or false), and areas of low signal caused by intravoxel dephasing can be seen with complex flow patterns associated with valvular stenosis or regurgitation ( Figure 20 ).
3
Flow Mapping
Velocity-encoded phase-contrast imaging can be used to quantify aortic flow. The phase-contrast technique is based on the fact that protons undergo a change in phase that is proportional to velocity when they pass through a magnetic field gradient consisting of equal pulses that are of opposite polarity and slightly offset in time. Blood flow can be quantified by integrating these measured velocities within the aortic lumen throughout the cardiac cycle with values that have shown strong agreement with phantom models and other measurement approaches. Phase-contrast imaging of the aorta can be used to assess forward flow and stenotic and regurgitant valves and can aid in assessment of congenital heart disease. Phase-contrast imaging is typically acquired in a single in-plane or through-plane direction, with some applications allowing flow encoding in multiple directions.
4
Contrast-Enhanced MR Angiography (MRA)
Contrast-enhanced MRA can provide a 3D data set of the aorta and branch vessels, allowing complex anatomy and postoperative changes to be depicted through postprocessing techniques such as maximum-intensity projection and multiplanar reformatting ( Figure 21 ). In patients with contraindications to contrast or in cases of difficult intravenous access, a 3D angiogram of the aorta can still be obtained with unenhanced segmented steady-state free precession angiography. When precise dimensions of the aortic root and proximal ascending aorta are needed, electrocardiographically gated techniques can be used. Improved scanning speed allows time-resolved MRA. Although contrast timing for contrast-enhanced MRA can be a challenge, particularly in the concurrent assessment of the aorta and pulmonary arteries or veins, the use of newer blood-pool contrast agents can circumvent the limitations of traditional interstitial gadolinium contrast agents and in conjunction with electrocardiographic and respiratory gating has been shown to increase vessel sharpness and reduce artifacts.
MRI may also be used as a tool to investigate aortic physiology. Quantification of stiffness, an important predictor of cardiovascular outcome, can be obtained with pulsewave measurements from high–temporal resolution cine imaging. MRI can provide insight into the elastic properties of the aorta, quantify the resultant blood flow, and estimate aortic wall shear stress.
5
Artifacts
Similar to echocardiographic imaging, MRI artifacts occur. Consequently, consistently recognizing artifacts can prevent misinterpretation. The reader is referred to two excellent reviews for a detailed discussion of these.
H
Invasive Aortography
Once considered the reference standard for the diagnosis of acute aortic diseases, invasive catheter-based aortography has largely been replaced by less invasive techniques, including CT, MRI, and TEE. These noninvasive imaging modalities provide higher sensitivity and specificity for detecting AAS and enable the assessment of aortic wall pathologies that are not seen on lumenograms (as obtained by contrast aortography). In addition, CT, MRI, and TEE also provide greater sensitivity in detecting supporting findings such as pericardial or pleural hemorrhage or effusion. Moreover, aortography is time consuming and incurs a risk for contrast-induced nephropathy. Thus, invasive aortography no longer has a role as a primary diagnostic modality for AAS.
Although invasive aortography has been replaced for diagnostic purposes, it continues to be useful to guide endovascular procedures and to screen for endoleakage. Intraprocedural contrast aortography is often essential to identify aortic side branches and provide important landmarks during the endovascular procedure. Figure 22 reveals the resolution of distal dynamic aortic obstruction after a stent graft was placed in a type B dissection. IVUS is an alternative imaging technique during endovascular procedures.
I
Comparison of Imaging Techniques
With advances in imaging technology, there are now multiple modalities well suited to imaging the thoracic aorta, including CTA, MRA, echocardiography, and aortography. No single modality is preferred for all patients or all clinical situations. Instead, the choice of imaging modality should be individualized on the basis of a patient’s clinical condition, the relevant diagnostic questions to be answered, and local institutional factors such as expertise and availability. A few pertinent comments follow.
When assessing broadly for the presence of thoracic aortic aneurysms (TAAs), or to size such aneurysms, CTA or MRA is preferred, as all segments of the thoracic aorta are well visualized. As well, the aorta and its branches can be displayed in multiple planar views, which permits more accurate diameter measurements than axial imaging. In addition, both modalities can provide a reconstructed, surface-shaded 3D display of the aorta, which is helpful in demonstrating the anatomic relations of the aorta and its branch vessels. In contrast, TEE is not generally preferred for routine aortic imaging, because it is semiinvasive, is relatively unpleasant for the patient, does not provide full visualization of the arch vessels, and does not permit easy identification of landmarks when comparing serial examinations to assess aortic changes over time.
When the region of clinical interest is specifically the aortic root, such as in screening for or following Marfan syndrome, TTE may be preferred, because the aortic root is generally well visualized and easily measured, whereas on conventional nongated CTA, the aortic root may be poorly visualized because of its angulation and significant motion artifact produced by the beating heart. On the other hand, echocardiography is less consistently able to image the distal ascending aorta, aortic arch, and descending thoracic aorta. To image these segments, CTA and MRA are preferred. Another consideration in selecting an imaging modality is the previous modality used. When following a patient with an enlarging aortic aneurysm, it is best to use the same imaging modality for future imaging, so that a comparison of one study with the next is comparing apples to apples rather than apples to oranges.
For imaging of suspected AAS, the primary consideration should be the accuracy of the imaging modality, given the serious consequences of false-positive and particularly of false-negative results. There have been a number of studies carried out over the past two decades comparing CTA, MRA, TEE, and aortography for the diagnosis of aortic dissection, and a recent meta-analysis by Shiga et al . showed that CTA, MRA, and TEE are all outstanding, with sensitivities of 98% to 100%, as shown in Figure 23 . On the other hand, aortography has a sensitivity of only 88%, perhaps reflecting the fact that IMH often goes undetected with this technique. In the same meta-analysis, the specificity of the four imaging modalities was roughly equivalent at 94% to 98%, as shown in Figure 24 . Therefore CTA, MRA, and TEE are all reasonable first-line imaging studies to choose for this purpose.
It is important to note, however, that the research studies that evaluate the accuracy of imaging modalities are usually performed at centers of excellence and interpreted by designated experts in aortic imaging, and it is therefore reasonable to suspect that accuracy may be lower when the same imaging modalities perform in the “real-world” setting. Indeed, a report from the International Registry of Acute Aortic Dissection (IRAD) examined this very question, and the results are shown in Figure 25 . The real-world sensitivity of both CTA and TEE is lower than in the above meta-analysis, probably reflecting a lesser degree of expertise among the readers. Interestingly, the real-world sensitivity of MRA remained at 100%, which may reflect the fact that MR angiograms tend to be read by specialists (e.g., vascular radiologists) rather than general radiologists (e.g., emergency department radiologists). The diagnostic and practical features of each of the five common imaging modalities are summarized in Tables 4 and 5 .
Diagnostic performance | CTA | TTE | TEE | MRA | Angiography |
---|---|---|---|---|---|
Sensitivity | +++ | ++ | +++ | +++ | ++ |
Specificity | +++ | ++ | +++ | +++ | +++ |
Ability to detect IMH | +++ | + | ++ | +++ | − |
Site of intimal tear | +++ | − | ++ | +++ | ++ |
Presence of AR | − | +++ | +++ | ++ | +++ |
Coronary artery involvement | + | − | ++ | + | +++ |
Presence of pericardial effusion | ++ | +++ | +++ | ++ | − |
Branch vessel involvement | ++ | − | + | ++ | +++ |
Advantages of modality | CTA | TTE | TEE | MRA | Angiography |
---|---|---|---|---|---|
Readily available | +++ | +++ | ++ | + | + |
Quickly performed | +++ | +++ | ++ | + | + |
Performed at bedside | − | +++ | +++ | − | − |
Noninvasive | +++ | +++ | + | +++ | − |
No iodinated contrast | − | +++ | +++ | +++ | − |
No ionizing radiation | − | +++ | +++ | +++ | − |
Cost | ++ | + | ++ | ++ | +++ |
III
Acute Aortic Syndromes
A
Introduction
The term AAS refers to the spectrum of aortic pathologies, including classic aortic dissection, IMH, penetrating aortic ulcer (PAU), and aortic aneurysm rupture (contained or not contained). Although the pathophysiology of these heterogeneous conditions differs, they are grouped because they share common features: (1) similar clinical presentation (“aortic pain”), (2) impaired integrity of the aortic wall, and (3) potential danger of aortic rupture requiring emergency attention. Moreover, some of these conditions may represent stages in the evolution of the same process. We have elected not to include, as some authors do, aortitis and traumatic aortic rupture, because they have totally distinct clinical and pathophysiologic profiles. Clinical databases, such as the IRAD, have contributed tremendously to our knowledge of these acute aortic pathologies.
Because of the life-threatening nature of these conditions, prompt and accurate diagnosis is paramount. Misdiagnosis of these conditions, usually because of confusion with myocardial ischemia, can lead to untimely deaths. Table 6 lists some less urgent conditions that can potentially mimic AAS.
Aortitis |
Atheromatous plaque |
Prior surgery of aorta |
Pericardial recess |
Remnant of a nonpatent PDA |
Artifacts on CT (streak and motion) |
Reverberation artifacts in ascending aorta on TEE |
Innominate vein |
Periaortic fat and hemiazygos sheath may mimic IMH |
The noninvasive imaging techniques that play a fundamental role in the diagnosis and management of patients with AAS include CTA, TTE, TEE and MRI. Some patients may require more than one noninvasive imaging study and, in rare instances, invasive aortography may be necessary. Imaging is used to confirm or exclude the diagnosis, determine the site(s) of involvement, delineate extension, and detect complications to plan the most appropriate management approach.
B
Aortic Dissection
1
Classification of Aortic Dissection
Accurate classification of aortic dissection is important because significant differences in clinical presentation, prognosis, and management depend on the location and extent of the dissection. Figure 26 illustrates the two commonly used classifications: the DeBakey system (types I, II, and III) and the Stanford system (types A and B). Dissections involving the aortic arch without involving the ascending aorta are classified as type B in the Stanford system. The majority of dissections, whether type A or type B, propagate beyond the diaphragm to the iliac arteries.
The appropriate management of aortic dissections depends not only on the location of the dissection but also on the time that has elapsed between onset of the process and the patient’s presentation. Although the adjectives acute , subacute , and chronic are often applied, there is no standard definition for these time periods. There is a 24-hour “hyperacute” period during which dissections involving the ascending aorta carry a risk for rupture approaching 1% per hour. Studies have shown that 75% of aortic dissection–related deaths occur in the initial 2 weeks. At the opposite extreme are “old dissections” encountered incidentally during aortic imaging or surgery. These are clearly “chronic.” Hirst et al ., Levinson et al ., and DeBakey considered an aortic dissection to be “acute” when the onset of symptoms was <2 weeks in duration at the time of diagnosis. The subsequent 2-month period was designated “subacute,” and beyond the second month, an aortic dissection was termed “chronic”. We endorse this classification, as it has some basis in pathologic observations. The extremely high initial death rate declines after 2 weeks. Moreover, friable aortic tissue extends beyond 2 weeks. By 6 to 8 weeks, the outer aortic wall has largely “healed,” in that it has developed scar, a reasonable marker for the beginning of the chronic stage. It must be acknowledged that any time-based division of “acute” from “subacute” and “subacute” from “chronic” is arbitrary. Nevertheless, such distinctions are necessary for analyzing outcomes. Moreover, some imaging features of acute and chronic dissections are different. In chronic dissection, the dissection flap tends to be thicker, more echodense, and relatively immobile (as distinct from the oscillating flaps seen in acute dissection).
2
Echocardiography (TTE and TEE)
The sensitivity of 2D TTE by fundamental imaging was previously reported to be only 70% to 80% for the detection of type A dissection. However, because of new transducers with improved resolution, harmonic imaging, and contrast enhancement, the sensitivity of TTE has improved to approximately 85% on the basis of recent data from Cecconi et al . and Evangelista et al . Therefore, TTE may be of some use as the initial imaging modality, especially in the emergency room ( Figure 27 ). In addition, TTE provides assessment of left ventricular contractility, pericardial effusion, aortic valve function, right ventricular size and function, and pulmonary artery pressure, which may facilitate the diagnosis of chest pain due to myocardial ischemia and/or infarction, pulmonary embolism, or pericardial disease and may identify dissection complications such as aortic regurgitation (AR) in an early fashion. Moreover, the use of contrast agents may further improve the accuracy, as illustrated in Figure 28 . Nevertheless, because of the potential catastrophic nature of type A aortic dissection, negative results on TTE should not be considered definitive, and further imaging should follow.
Moreover, TTE is less sensitive for the diagnosis of type B dissection, because the descending thoracic aorta (located farther from the transducer) is imaged less easily and accurately. Therefore, although TTE may be diagnostic in many instances, its role is predominantly that of a screening procedure. TEE, on the other hand, is highly accurate for establishing the diagnosis of both type A and type B acute aortic dissection. Since the landmark multicenter European Cooperative Study, several additional studies have demonstrated the high accuracy of TEE, with sensitivity approaching 100%.
a
Echocardiographic Findings
The diagnostic hallmark of aortic dissection is a mobile dissection flap that separates the true and false lumens ( Figure 29 ). Important features of the dissection flap include oscillation or motion that is independent of the aorta itself, visualization in more than one view, and clear distinction from reverberations from other structures, such as a calcified aortic wall, catheter in the right ventricular outflow tract, pacemaker wire, or pericardial fluid in the transverse oblique sinus.
The true and false lumens can almost always be differentiated. In the descending thoracic aorta, the false lumen is usually larger than the true lumen. The dissection flap typically moves toward the false lumen in systole (systolic expansion of the true lumen) and toward the true lumen in diastole (diastolic expansion of the false lumen), sometimes causing compression of the true lumen. Moreover, the characteristics of blood flow vary in the true and false lumens. In the true lumen, antegrade systolic flow is rapid enough to create brighter shades of red or blue on color Doppler. In contrast, flow in the false lumen is generally slower, producing duller colors. In fact, flow in the false lumen may be absent or in the opposite direction (retrograde) to that of the true lumen. The sluggish flow in the false lumen may result in the presence of spontaneous echo contrast, sometimes referred to as “smoke.” The false lumen may also contain variable degrees of thrombus. Additional findings in patients with aortic dissection include dilatation of the aorta, compression of the left atrium, AR, pericardial and/or pleural effusion, and involvement of the coronary arteries. Table 7 summarizes the main echocardiographic findings in aortic dissection. Three-dimensional TEE may provide information beyond what can be obtained with 2D TEE. For example, the size of the entry tear size and its relationship to surrounding structures may be shown in greater detail, allowing better morphologic and dynamic evaluation of aortic dissection ( Figure 30 ). Such information may be particularly helpful when the flap spirals around the long axis of the aorta. Moreover, 3D TEE demonstrates the dissection flap not as a linear structure but as a sheet of tissue of variable thickness in the long, short, or oblique axis. This may make it possible to distinguish a true dissection flap from an artifact when it is relatively immobile. In addition, multiplane 3D TEE provides a more rapid and accurate evaluation of the aortic arch than 2D TEE.
Diagnostic goals | Definition by echocardiography |
---|---|
Identify presence of a dissection flap | Flap dividing two lumens |
Define extension of aortic dissection | Extension of the flap and true/false lumens in the aortic root(ascending/arch/descending abdominal aorta) |
Identify true lumen | Systolic expansion, diastolic collapse, systolic jet directed away from the lumen, absence of spontaneous contrast, forward systolic flow) |
Identify false lumen | Diastolic diameter increase, spontaneous contrast and or thrombus formation, reverse/delayed or absent flow |
Identify presence of false luminal thrombosis | Mass separated from the intimal flap and aortic wall inside the false lumen |
Localize entry tear | Disruption of the flap continuity with fluttering or ruptured intimal borders; color Doppler shows flow through the tear |
Assess presence, severity and mechanisms of AR | Anatomic definition of the valve (bicuspid, degenerated, normal with/without prolapse of one cusp); dilation of different segments of the aorta; flap invagination into the valve; severity by classic echocardiographic criteria |
Assess coronary artery involvement | Flap invaginated into the coronary ostium; flap obstructing the ostium; absence of coronary flow; new regional wall motion abnormalities |
Assess side-branch involvement | Flap invaginated into the aortic branches |
Detect pericardial and/or pleural effusion | Echo-free space in the pericardium/pleura |
Detect signs of cardiac tamponade | Classic echocardiographic and Doppler signs of tamponade |
b
Detection of Complications
AR occurs in approximately 50% of patients with type A aortic dissection. The presence, severity, and mechanism(s) of AR may influence surgical decision making and aid the surgeon in deciding whether to spare, repair, or replace the aortic valve. The mechanisms of AR are listed in Table 8 , and several of these are illustrated in Figure 31 . These mechanisms will be discussed in greater detail in section III.B.6, “Use of TEE to Guide Surgery for Type A Aortic Dissection.”
|
A pericardial effusion in an ascending aortic dissection is an indicator of poor prognosis and suggests rupture of the false lumen in the pericardium. Echocardiography is the best diagnostic technique for estimating the presence and severity of tamponade. Periaortic hematoma and pleural effusion are best diagnosed by CT. The presence of periaortic hematoma has also been related to increased mortality.
TEE is capable of imaging the ostia and proximal segments of the coronary arteries in nearly all patients and may demonstrate coronary involvement due to dissection (flap invagination into the coronary ostium and origin of coronary ostium from the false lumen). Color Doppler is useful for verifying normal or abnormal or absent flow into the proximal coronary arteries. Detection of segmental wall motion abnormalities of the left ventricle by TTE or TEE may also help identify this complication. Color Doppler also reveals reentry sites (often multiple, as in Figure 32 ), which explain why the false lumen often remains patent over time.
c
Limitations of TEE
The limitations of TEE for evaluating patients with aortic dissection are few but deserve mention. Interposition of the trachea between the ascending aorta and the esophagus limits visualization of the distal ascending aorta and proximal arch. In a small number of patients, the dissection may be limited to this area, making detection more difficult. In addition, the cerebral vessels (especially the brachiocephalic and left common carotid arteries) can be difficult to image by TEE. Moreover, the celiac trunk and superior mesenteric artery cannot be consistently imaged by TEE, and CT is considered the gold standard for detecting complications below the diaphragm. Last, TEE depends largely on operator skill for image acquisition and interpretation. Reverberation artifacts, especially in the ascending aorta, can mimic a dissection flap and result in a false-positive diagnosis. Knowledge of mediastinal and para-aortic tissues (e.g., the hemiazygos sheath, the thoracic venous anatomy and common anatomic variants) is essential.
3
CT
Data from the IRAD published in 2000 showed that among 464 patients with acute aortic dissections (62% with type A), nearly two-thirds underwent CTA as the initial diagnostic imaging. The computed tomographic data in this study were acquired on older generation scanners, which may explain the fact that most patients underwent several imaging tests (average, 1.8 tests).
A more recent IRAD publication, now including 894 patients, showed that the “quickest diagnostic times” were achieved when the initial test was CT, whereas the initial use of MRI or catheter-based aortography resulted in significantly longer diagnostic times.
Today, newer generation modern multidetector computed tomographic scanners are ubiquitous even in remote-area hospitals throughout the United States and Europe and are usually staffed and readily available 24 hours a day. In 2007, according to 2011 health data from the Organisation for Economic Co-operation and Development, there existed 34.3 computed tomographic scanners per million population in the United States, and 185 computed tomographic examinations were performed per 1,000 patients in US hospitals.
Computed tomographic angiographic protocols are robust and relatively operator independent. Computed tomographic angiographic protocols that are designed to exclude dissections typically begin with low-dose noncontrast CT to exclude the possibility of IMH, followed by contrast-enhanced computed tomographic angiography. The coverage includes the entire thorax, abdomen, and pelvis to allow delineation of the extent of a flap and its extension into branch vessels and to evaluate for end-organ ischemia (e.g., bowel or kidneys), and possible extravasation. Examples of computed tomographic angiography are illustrated in Figures 33 and 34 .
Diagnostic accuracy is extremely high for the exclusion of aortic dissection (98%–100%). However, false positives for the detection of type A dissection near the aortic arch may infrequently occur with older generation computed tomographic scanners, which may lead to unnecessary operations. Single-slice spiral computed tomographic scanners and early-generation multidetector computed tomographic scanners frequently demonstrate pulsation artifact in the ascending aorta, which occasionally may mimic type A dissection (pseudoflaps). However, aortic pulsation artifact and pseudoflaps can be completely eliminated with the use of electrocardiographically gated computed tomographic angiographic acquisitions. Therefore, it is advisable to use electrocardiographic gating or triggering if ascending aortic pathology is suspected. False-positive results on CT leading to unnecessary surgery for aortic dissection have not been reported to date with the use of newer generation electrocardiographically gated multidetector computed tomographic angiographic scans.
Surgery or transcatheter intervention in type B dissection may be indicated if there is occlusion of major aortic branches leading to end-organ ischemia or expansion of the aortic diameter or interval extension of the dissection flap. MDCT allows imaging of the entire aorta and iliac system within seconds and allows delineating the intimal flap extension into aortic arch vessels and the abdominal aorta and its branches as well as the iliac system, which may determine the feasibility of stent-graft repair. Entry and reentry sites, aortic diameters, and the relationships between true and false lumen can be defined using multiplanar multidetector computed tomographic reformations. MDCT also allows the determination of end-organ perfusion, such as asymmetric or absent enhancement of kidneys in case of renal artery occlusion.
Given the multiplanar reformation capabilities that, unlike MRI, can be applied post hoc, and 3D imaging capabilities, CT has extremely high retest reliability for measurement of aortic diameters on follow-up scans. The multiplanar reconstruction capabilities facilitate endovascular treatment planning and may allow the determination of proximal fenestrations that may be amenable to endovascular repair. Because determination of these features is important, reporting of the extension of dissection and aneurysms into branch vessels and secondary end-organ hypoperfusion are considered “essential elements” of aortic imaging reports. Gated MDCT may determine proximal extent of the flap into coronary artery ostia, or the aortic valve, as well as presence of pericardial effusion or hemopericardium.
Gated MDCT may simultaneously exclude the presence of obstructive coronary artery disease in acute dissections, as well as coronary artery dissection and aortic valve tears. In addition, combination of a gated or triggered thoracic computed tomographic angiographic acquisition with a nongated abdominal and pelvic acquisition is feasible at low radiation doses.
Further dose reduction using axial prospective electrocardiographic triggering (compared with spiral retrospective gating) computed tomographic angiography at a tube potential of 100 kV allows the further reduction of radiation doses without impairment of image quality of the aorta or coronary arteries.
The “triple rule-out” protocol for assessing acute chest pain in the emergency room is rarely needed and is neither technically suitable nor medically necessary on a routine basis. Optimal protocols for coronary CT angiography, for pulmonary embolism, and for aortic dissection differ, and “triple rule-out” CT is not optimal for all three. Given the increased radiation and contrast exposure and the lack of accurate diagnostic data for aortic dissection, there are no grounds to recommend triple rule-out CT for this condition. If there is a reasonable clinical suspicion for aortic dissection, then the highest quality study for this specific indication should be performed.
In summary, CT angiography is readily available throughout the United States and Europe; is most often the first imaging test when acute aortic dissection is suspected; has extremely high diagnostic accuracy; allows the evaluation of the entire aorta and its branches, the coronary arteries, the aortic valve, and the pericardium; and results in the shortest time to diagnosis compared with other imaging modalities, therefore allowing rapid initiation of therapy. Disadvantages of CT include the need for iodinated contrast material and ionizing radiation, although substantial dose reductions have recently been achieved with newer hardware technology and imaging protocols, and this issue may be of less concern in the setting of AAS.
4
MRI of Aortic Dissection
Early identification of aortic dissection and precise characterization of anatomic details are critical for clinical and surgical management of this condition. Imaging of suspected dissection should address not only the presence of a dissection flap and its extent but also the entry and reentry points, presence and severity of aortic insufficiency, and flow into arch and visceral branch vessels. MRI, which can address all of these issues noninvasively, provides high spatial and contrast resolution and functional assessment with an imaging time of 20 to 30 min. Specifically, MRI has very high sensitivity (97%–100%) and specificity (94%–100%) for diagnosing dissection. MRI also provides imaging without the burden of ionizing radiation, an important consideration for patients who undergo serial assessments of a known aortic dissection.
MRI does have potential limitations in this patient population. Although the scan times for MRI are relatively short, they are significantly longer than the scan times for CT angiography. Additionally, physiologic waveforms are challenging to obtain within the MRI scanner environment. Although cardiac rhythm, blood pressure, and oximetry can be monitored with MRI-appropriate equipment, caring for patients within an MRI scanning area can be difficult in emergent or unstable clinical scenarios that may be associated with aortic dissection.
A combination of dark-blood and bright-blood images in axial and oblique planes oriented to the aorta allows the detection and characterization of intimal flaps. True and false lumens can be differentiated by patterns of flow and by anatomic features ( Figures 35 and 36 ). The false lumen can often be identified on spin-echo images by a higher intraluminal signal intensity attributable to slower flow and may be characterized by web-like remnants of dissected media. Cine bright-blood imaging can also be used to directly visualize flow patterns within true and false lumens. Associated anatomic findings outside of the aorta on MRI may also be of interest, such as high signal intensity within pericardial effusion on dark-blood imaging, indicating the possibility of the ascending aorta rupturing into the pericardial space. Phase-contrast imaging can provide flow quantification of aortic insufficiency associated with dissection and can also allow definition of entry and reentry sites and differentiation of slow flow and thrombus in the false lumen. Newer 3D phase-contrast approaches have shown promise in further defining the flow characteristics and associated parameters of aortic dissection, such as wall stress.
Contrast-enhanced 3D MRA provides 3D data, results that allow postprocessing and detailed assessment of aortic and large-branch vessel anatomy in cases of dissection. The dynamics of aortic flow can also be evaluated with time-resolved MRA. Imaging with blood-pool contrast agents allows steady-state phase scanning, which can improve spatial resolution and better demonstrate the amount of thrombus within the false lumen.
5
Imaging Algorithm
Aortic dissection is a life-threatening condition that is associated with high early mortality and therefore requires prompt and accurate diagnosis. Numerous publications have sought to establish the relative merits of CT, TEE, and MRI as first-line imaging modalities. In truth, each diagnostic method has its strengths and weakness, as previously discussed. The optimal choice of imaging modality at a given institution should depend not only on the proven accuracy (all three are highly accurate) but also on the availability of the techniques and on the experience and confidence of the physician performing and interpreting the technique. CT has become the most commonly used first-time imaging modality partly because it is more readily available on a 24-hour basis. TEE may be the preferred imaging modality in the emergency room, if an experienced cardiologist is available, because it provides immediate and sufficient information to determine if emergency surgery will be required. Although CT may be less accurate for determining the degree and mechanism of AR, this can be evaluated by TTE and/or intraoperative TEE. The relative advantages and disadvantages of the various imaging modalities are summarized in Table 9 .
Modality | Recommendation | Advantages | Disadvantages |
---|---|---|---|
CT | First-line |
|
|
TEE | First- and second-line |
|
|
TTE | Second-line |
|
|
MRI | Third-line |
|
|
Angiography | Fourth-line |
|
|
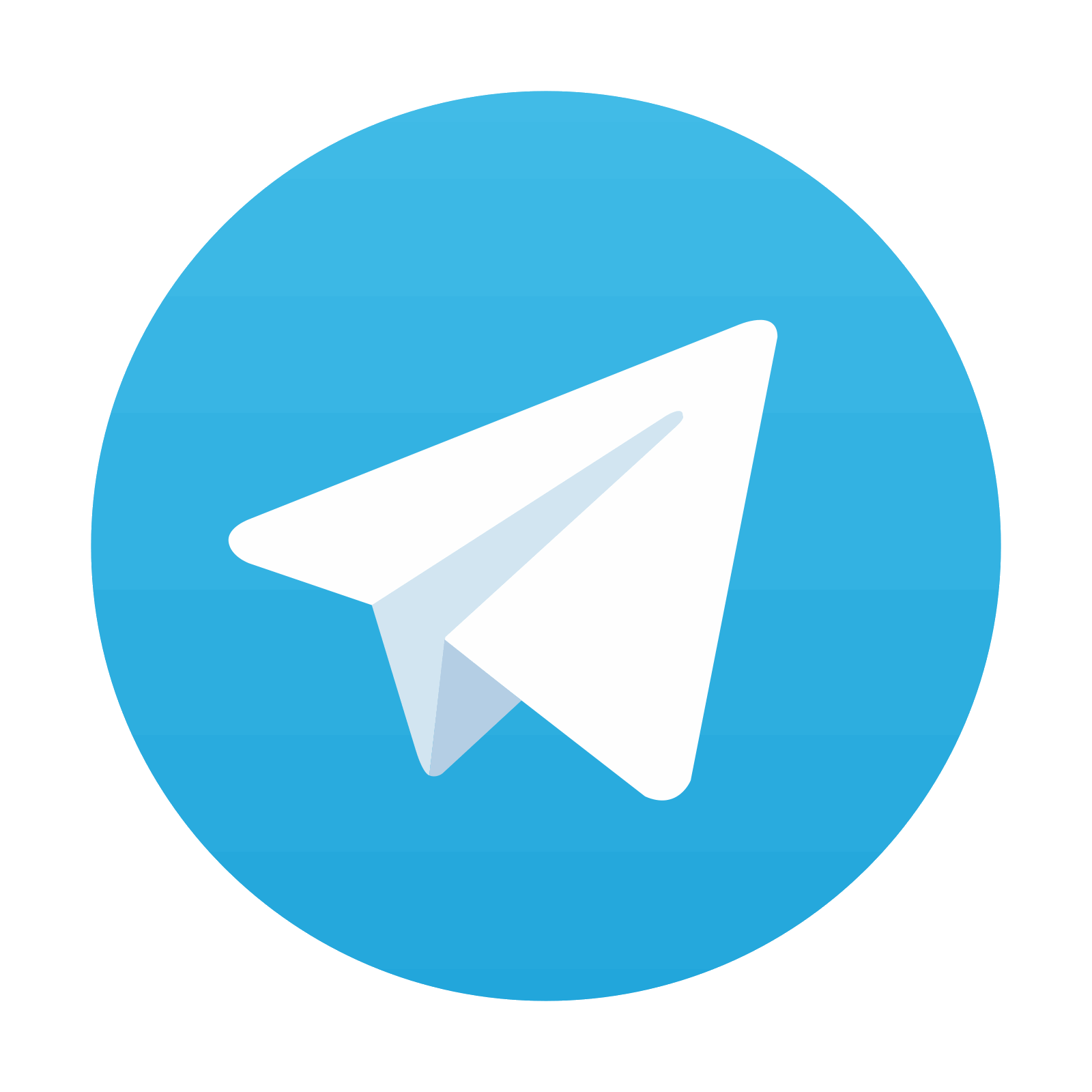
Stay updated, free articles. Join our Telegram channel
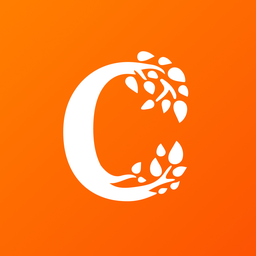
Full access? Get Clinical Tree
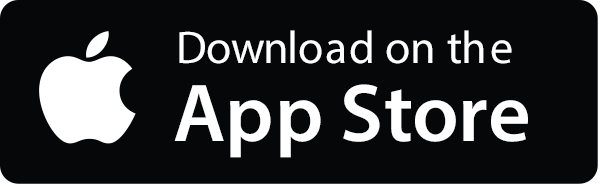
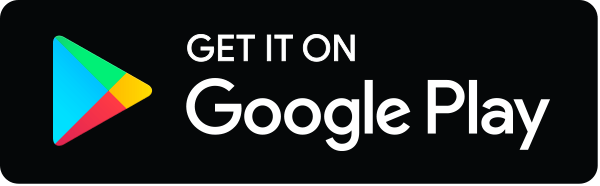
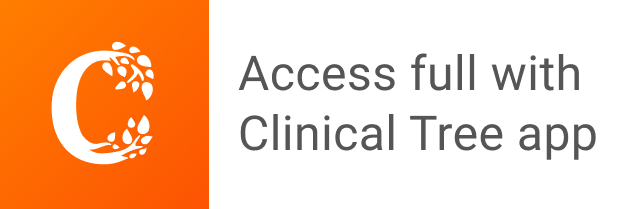