Year
1982
1987
1997
2003
NSR field strength
2 T
2 T
4 T
8 Ta
MR Injuries and Deaths
MRI is now a common procedure and is practiced on a worldwide basis. As a result there is no certain way to enumerate the number of deaths possibly related to MRI scanning. However, the known instances are extremely rare. As MRI is almost always performed in technically advanced locations with good access to communication networks it seems unlikely that there would be large numbers of unreported cases. A brief literature review in 1998 found reports of seven deaths attributed, at least in part, to MR scanning [62, 29], though in the absence of thorough clinical histories, autopsy results and details of scanning procedures in what were often gravely ill patients, it is uncertain whether MRI played a dominant role in any of these fatal outcomes.
However, two cases have been described in some detail and are worth careful study for the insight they provide regarding the type of circumstances that can rapidly lead to catastrophic outcomes. One is an example of magnetic foreign bodies and the other is an example of the missile effect.
In 1992 a 74-year old woman was referred to a major medical center in San Antonio, Texas for an MRI study [41]. The screening questionnaire disclosed that she had received an intracranial aneurysm clip at another institution in 1978. As a result the scan was not performed. However, the patient and her family returned in a few days and indicated they had contacted the operating neurosurgeon at the previous institution and had obtained the details of the implanted clip. This clip was identified as non-magnetic in published lists of medical devices and the MRI study was rescheduled. At the time of scanning, when approximately four feet from the 1.5 T magnet the patient complained of severe headache and her condition rapidly deteriorated. The patient was intubated and an emergency computed tomography study showed a large hematoma with subarachnoid hemorrhage. The patient died on the next day. Autopsy disclosed the clip and an arterial tear at the site of the clip placement. Study of the clip showed that it was not of the type that had been indicated. Instead, the actual clip was listed as magnetic and this was verified by testing in the 1.5 T magnet.
In July 2001 a 6-year-old boy was operated on for a benign brain tumor at a large medical center near New York City. After the procedure he was taken to the MRI facility for postoperative imaging. He developed respiratory difficulty while in the scanner. The two technologists performing the scan were temporarily distracted because of difficulties with a built-in oxygen supply. As an emergency measure another hospital employee brought a ferromagnetic portable oxygen tank into the room. The tank was drawn rapidly into the magnet striking in boy in the head and fracturing his skull. The boy died 2 days later [2, 47]. This case provoked much discussion about how to further improve the safety of MR imaging with a focus on staff training, architectural design of imaging suites to give technologists better control over people and items entering the scan room, and the advantages and disadvantages of ferromagnetic detectors at scan room entrances.
As mentioned above, well over one-half billion MRI scans have been completed safely since the introduction of the technique. However, these two tragedies indicate the need for continual vigilance and improvements in operating procedures, training, and site design. They also emphasize how rapidly the interaction between powerful magnetic fields and ferromagnetic materials can produce a catastrophe.
Static Magnetic Fields
The essence of an MRI scanner is a large, powerful magnet used to partially align the magnetic moments of the protons within the patient’s body against the forces of thermal randomization and to drive their precessional motion. These magnets can be constructed using various techniques, but the majority of clinical MRI scanners are built using a series of four to eight circular coils of wire on a common axis. This gives the scanner the form of an open circular cylinder that must be large enough to completely and comfortably surround the patient being imaged. These coils have a diameter of approximately 1.0 m. Depending on the desired field strength, a scanner may use a total of between 2 and 400 km of wire conductor. In addition to the requirement for an intense strength, it is necessary that the magnetic field be very uniform over the imaging volume – on the order of 1.0 part-per-million (ppm) – and very stable – on the order of 0.1 ppm/h – over the time of the imaging process. In order to achieve this high level of field homogeneity the coils must be wound and positioned to a very high level of accuracy. The main-field magnet constitutes most of the weight and cost of the scanner.
In the late 1970s the earliest whole-body MRI magnets used aluminum or copper wire as the conducting material. These resistive materials heat up substantially when carrying the large electric currents required to achieve strong magnetic fields. Consequently, these resistive magnets were limited to producing imaging fields on the order of 0.05–0.3 T. In the early 1980s the introduction of whole-body superconducting magnets using niobium-titanium alloy wire permitted much higher field strengths, most commonly 1.5 T, and consequently much improved imaging performance. In order to maintain the superconducting state it is necessary to maintain the coils at a temperature close to absolute zero, typically 4.2 K. This necessitates enclosing the coils in a vacuum-walled cryostat that contains a bath of liquid helium.
Gradient Fields
Specially designed coils are used to produce gradients in the magnetic field strength across the region of imaging. There are generally three separate coils – one for each of the Cartesian spatial coordinates, x, y and z. The strength of each of these gradient fields is switched between various levels during the imaging process. Each of these coils generally consists of a layer of a specific conductor pattern mounted on a cylindrical coil form. The completed cylindrical gradient package is located between the patient being imaged and coils of the main magnet. A shield gradient coil is usually placed between the primary gradient coils and the static-field magnet in order to minimize the interaction of this magnet with the gradient coils and to limit the spurious gradient fields produced by eddy currents induced in the electrically conducting components of the scanner.
The strength of the gradient fields determines the spatial resolving power of the scanner and its ability to measure diffusion of water molecules in the patient’s tissues. Currently available MRI scanners generally are capable of producing gradient strengths on the order of 10–100 mT/m. An important capability of the gradient system is the slew rate (SR), which is a measure of the time required to switch the gradient strength from one level to another. The SR is determined both by the properties of the gradient coil and of the power supply used to drive it. The SR in clinical MRI scanners can range up to approximately 200 mT/m/ms in whole-body systems and can be several times higher than this in systems using small local gradient coils used to image a specific region of the body.
Peripheral Nerve Stimulation (PNS)
The magnetic fields produced by the gradient coils are much weaker than the main static field and create no additional safety issues. However, the electric fields produced when the gradient currents are time-dependent can interact with the nervous system to produce sensations that may, in some cases, be unpleasant. These sensations are usually viewed as the result of a cascade of phenomena: the time-dependent coil currents produce electric fields which induce electric current densities in the tissues; if the amplitude is sufficient and the direction of the induced currents are parallel to relevant peripheral nerve cells they will depolarize the axons of those cells, leading to action potentials propagating into the central nervous system (CNS). Here they will be perceived as some form of sensation arising at the location of the depolarization [10]. Qualitatively, the production of sensations by coil current pulses is quite straightforward. However, attempts to treat the phenomenon quantitatively in a practical imaging situation require complex models involving the details of the coil geometry, the pulse sequences and switching parameters being used, the geometry and electrical properties of the involved body region and the nerve networks in this region. Several models based on the cable theory of nerve excitation have been developed for specific aspects of this process [13, 57, 86]. These models can provide qualitative guidelines for designing coils and pulse sequences to minimize the effects of PNS, but experimental investigation is necessary to achieve quantitative understanding.
It does not appear that a single depolarization pulse is ordinarily sufficient to create a perceived sensation in the CNS; instead, a sequence of several closely spaced pulses is usually required. For example, in one study, PNS thresholds were studied by use of a sequence consisting of 256 trapezoidal current pulses each approximately 1.3 ms long with the sequence repeated once every second [22]. Thresholds for excitation by a single pulse would be expected to be very much higher. Currents flowing parallel to nerve axons are much more effective in creating PNS than those flowing perpendicular to them.
The initial approach to regulation of gradient switching attempted to put a quantitative limit on the amplitude and duration of the magnetic fields produced by gradient coils. For example an early FDA regulation put an upper limit of 20 T/s for switching pulses lasting more than 0.12 ms [5]. This approach proved unwieldy and quite restrictive on scanner performance. Later it was recognized that nerve excitation per se is not harmful and it is only necessary to assure that PNS does not lead to painful sensations. For that reason the current FDA guidelines use a pragmatic criterion, requiring that the threshold for significant risk is “any time rate of change of gradient fields (dB/dt) sufficient to produce severe discomfort or painful nerve stimulation”.
It is important to note that although PNS is often cited as a serious hazard of MRI, the same process of using coils carrying time-dependent currents to depolarize cells in the nervous system is used in procedures such as transcranial magnetic stimulation (TMS) [8], which are generally not considered hazardous and potentially therapeutic. In TMS the induced electric currents in the body are orders of magnitude larger than those produced in any MRI situation. TMS is being explored as a treatment for depression and motor disorders of the CNS [1, 15]. Thus, it appears that PNS is unlikely to produce serious injury in MRI. It can be ameliorated when necessary by reducing the number and amplitude of the gradient switching events in the pulse sequence.
Acoustics
Because the gradient coils are located within the strong static magnetic field of the main magnet, the pulsing of current through these coils during the MRI exam causes time-dependent Lorentz forces, resulting in sometimes-high acoustic noise levels. Indeed, the magnet/gradient system is in many respects similar to a dynamic loudspeaker system, which uses much smaller magnets and coils to convert electrical signals into sound. Sound pressure levels can reach 118 dBA or higher during an MRI exam [55], depending on the main magnetic field strength and the specific imaging pulse sequence.
The acoustic noise generated during the MRI exam can cause a variety of problems for patients and healthcare workers, ranging from increased anxiety and difficulties in communication to temporary or even permanent hearing loss. For this reason, hearing protection in the form of headphones or disposable earplugs is required. These measures can reduce the sound pressure levels experienced by the patient by up to roughly 30 dB [70]. Various government agencies have developed limits for sound level exposure for patients and workers, above which hearing protection should be worn. In the UK, the Medical Device Agency (MDA) recommends limits of 85 dBA averaged over 8 h for patients, or 100 dBA averaged over 15 min [55]. In the US, the FDA considers MRI studies to have significant risk if peak unweighted sound pressure levels reach 140 dB or A-weighted levels exceed 99 dBA with hearing protection in place [19]. The Occupational Safety and Health Administration (OSHA) workplace noise regulations limit noise exposures to 90 dBA averaged over an 8-h day, or 110 dBA over 30 min [52].
Radiofrequency Heating
The radiofrequency (RF) B 1 field used to manipulate the axis of spin rotation is inevitably accompanied by an electric field E, and this field, in turn, produces a current density J, within the patient’s tissues. Using complex number notation, J can be written as the sum of a real component, J r = σE, in phase with E and an imaginary component, J i , 90° out-of-phase with E. Here σ is the conductivity of the patient’s tissues at the Larmor frequency.
The induced current density in the patient increases strongly with field strength and frequency and has profound implications for high-field MRI. In particular, the in-phase component of J, acting through the Joule heating effect, produces an energy deposition in the tissues given by
. This energy deposition tends to heat the tissues and is measured by the specific absorption rate (SAR). During most pulse sequences, the RF energy is supplied in a series of short bursts and therefore the RF energy is not deposited continually during imaging but as a series of short pulses. The total heating effect of an imaging sequence depends on the strength, E, and frequency of the applied electric field and the duty cycle (fraction of the time the transmitter field is on). The FDA provides guidance [19] as to whether patient heating from a given sequence should be considered as a significant risk when the whole-body SAR averaged over a 15-min examination is greater than 4 W/kg. Similarly, the local SAR in the head is considered as significant risk if it larger than 8 W/kg when averaged over an 8 min examination.

In addition to the above global heating effects associated with the B1 field, a number of other potential safety issues are associated with the RF transmitter fields. For example, the transmitter and receiver coils used in MRI contain tuning capacitors in series with the conducting loops of the coils. During RF transmission these capacitors may develop a large RF voltage in their immediate vicinity. These local voltages have the potential to produce local RF burns if the patient’s skin is too close to them. For this reason, RF coils, particularly surface RF coils, are constructed with insulating coverings to prevent close contact of these capacitors with the patient’s skin. Care should be taken to prevent accidental contact during the scanning procedure.
There may be a risk of RF heating from any external conducting devices that are in proximity to the patient [72]. All non-essential electrically conductive materials should be removed from the MR system before scanning. Care must be taken with the use of any ECG gating systems. Conventional ECG electrodes and leads are not classified as MR safe because of their incorporation of low-impedance conductors and/or ferromagnetic components, which can cause skin heating or burns. Only MR-safe electrodes and leads should be used; these can be found in the MR vendors’ accessories catalogues. Care should also be taken with placement of ECG electrodes and leads. Leads should not be allowed to form loops, and should not be positioned along the sides of the scanner bore or close to the body RF coil or any other RF transmit coil [72].
Local edema and tissue swelling as well as localized image artifacts have been noted during MRI of patients with tattooing or permanently implanted eye shadow. This effect has been attributed to an interaction of the radiofrequency field with electrically conducting components of the implanted pigments [46, 64, 81]. Also, medication patches containing metallic fibers or backing [21] are potential sources of RF-induced patient injury.
Patient Monitoring
While ECGs are used routinely for pulse sequence gating or triggering in cardiovascular MRI exams, their use for patient monitoring in this setting is problematic, in part because of the magneto-hydrodynamic (MHD) effect. This effect occurs when charged ions in flowing blood move though the magnetic field, inducing a voltage that can distort the ECG trace, especially in scanners with higher magnetic field strengths. This effect is especially prominent during the ST segment of the ECG during systolic ejection of blood, and thus can mask ST depression or elevation related to myocardial ischemia. In fact, the T wave can be elevated to a higher level than the R wave, possibly interfering with MRI gating. Figure 8.1 compares the ECG waveform recorded from a subject outside vs. inside the bore at 3.0 T, with elevated T-wave inside the bore due to the MHD effect. Fortunately, modern scanners equipped with vectorcardiogram (VCG) gating can offer more reliable detection of the R wave compared to conventional ECG gating by exploiting spatial information in the VCG. Additional distortion of the ECG waveform can be induced by radiofrequency pulses and gradient switching during MRI. Therefore, the ECG waveform acquired during MRI should only be regarded as a general representation rather than an exact depiction of the patient’s cardiac electrical activity and should not be used for diagnostic purposes.
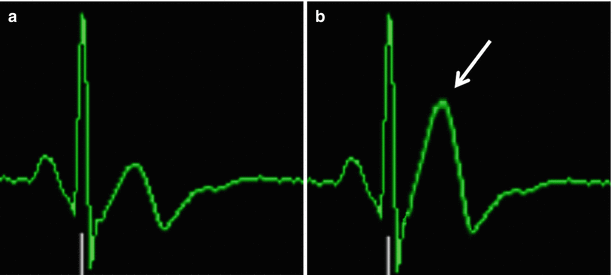
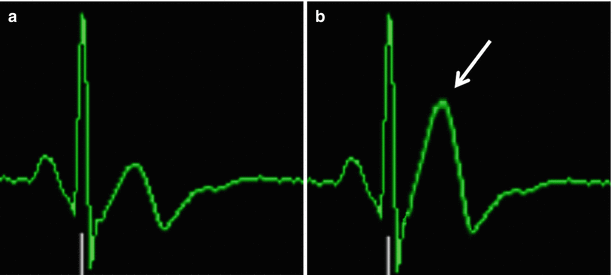
Fig. 8.1
ECG waveforms recorded from the same subject lying outside (a) vs. inside (b) a 3.0 T scanner bore. Note the T wave elevation in (b) (arrow) caused by the magneto-hydrodynamic effect
Physiological and Sensory Effects
Since high-field MRI magnets first became available, some patients undergoing MRI have reported a low level of specific physiological and sensory effects. These effects ordinarily do not represent true safety hazards but in some cases may be somewhat uncomfortable. They are generally associated with motion in the magnetic field or exposure to time-dependent magnetic fields – processes that are associated with low level induced electric fields within the tissues. These effects include metallic tastes, magnetophosphenes, balance disturbance, dizziness, vertigo and nausea [61, 63]. Importantly, these symptoms generally cease immediately or shortly after the subjects leave the vicinity of the magnet and are reported only by a minority of the subjects studied. Frank vomiting and incapacitating disturbances of gait or balance disturbances are rarely, if ever, reported. There is an impression that there are some individuals who are much more sensitive to these effects than is the norm, although this possibility has apparently not been studied quantitatively.
As one example, sensations of metallic taste have been reported with head motions in high field scanners [61]. One study [12] has measured the thresholds for the necessary rate of head motion and has found that the effect does not require the presence of dental fillings. It is likely that these metallic tastes result from motion-induced electric currents acting to depolarize sensory cells or their associated nerve cells.
Phosphenes are defined as visual sensations that occur even with no light entering the eye. When produced by an electric circuit with leads attached to the head in the vicinity of the eye they are called electrophosphenes; when produced by oscillating currents in a non-contacting electric coil they are called magnetophosphenes [26, 44, 45]. In high field MRI scanners in darkened rooms, brief sensations of faint light flashes are often reported when the direction of gaze is rapidly changed [61] and it has also recently been reported when subjects are exposed to very strong, rapidly switched gradient fields [68]. Magnetophosphenes are attributed to nerve or sensory cell depolarization in the optic nerve or retina or caused by electric currents induced by head or eye motion of in the applied magnetic field. Magnetophosphenes thus appear to be analogous to the sensations associated with PNS but the depolarized electrically excitable tissue here is in the visual apparatus rather than the peripheral nervous system.
Low grade and transient balance disturbances, dizziness, vertigo and nausea sometimes reported during and immediately following exposure to high field magnets has generally been attributed to magnetic field interactions with the vestibular system and semicircular canals of the inner ear [61, 66]. A significant advance in the understanding of this effect resulted from a report that in high-field systems (3 and 7 T) in darkened rooms, a robust, spontaneous nystagmus develops in patients when lying still within the magnet [58, 77]. Infrared video cameras were used to observe this effect and demonstrated that it persists throughout the field exposure and does not require head or other motion. This nystagmus is attributed to a steady excitation of the vestibular portion of the eighth cranial nerve produced by a Lorentz force between the applied magnetic field and a constant electric current in the endolymphatic fluid within the inner ear. The reported vertigo, nausea and balance disturbances are all low level effects and do not represent a patient safety issue. They are most likely explained by the conflict hypothesis, wherein the brain is presented with incompatible positional information from the visual and vestibular systems resulting in the symptoms of motion sickness [51, 79].
As imaging systems operating at 7 T or higher (research only) have become more common over the last decade, reports both anecdotal and in the literature have indicated that a fraction of subjects and system operators exposed to them have reported a significant level of discomfort [30, 80]. The symptoms are similar, but generally milder, than those of car sickness, sea sickness, air sickness and space sickness, i.e., the symptoms of motion sickness, which are also explained by the conflict hypothesis. It has been suggested that the term ‘magnet sickness’ be used for the magnetic field-induced symptoms [65]. It is important to emphasize that, at present-day MRI field strengths, these symptoms are quite mild, are experienced by only a minority of subjects, and do not significantly limit the utility of these scanners. However, at still higher field strengths the symptoms may become stronger and affect a larger fraction of the population. This explains the widespread practice of moving subjects slowly in high field magnets and it also raises the possibility that subjects prone to this discomfort might benefit from the use of medications used in the treatment of motion sickness including dimenhydrinate (Dramamine), meclizine (Antivert) and ginger root.
Lymphocyte Studies
It has recently been reported that blood lymphocytes from human venous samples taken before and after 1.5 T cardiac MRI demonstrated significant but transient increases of micronuclei [75] and increases in double-strand DNA breaks after MRI [23]. However, the significance of these studies for human exposure to cardiac MRI is uncertain and it has been noted that the lymphocyte DNA studies are subject to a variety of confounding factors [54].
Cardiac MR in Patients with Cardiovascular Devices
Patient Screening
In patients being referred for cardiac MR (CMR) examination, there is increasing prevalence of implants of various kinds, including passive and active cardiovascular devices [42]. Therefore it is especially important that every patient receive thorough screening before undergoing MR examination. This should include an interview with a licensed technologist trained in MR safety, supervised by a physician knowledgeable in CMR, and completion of a standardized MR screening form. Examples of these forms can be downloaded from a number of websites (MRI Safety Web Site, Expert Panel on MR Safety 2013 – Appendix 2). Whenever possible, the specific type of any implanted device should be confirmed by use of wallet cards that may have been issued to the patient, or by procedure notes in the patient’s records. If an unfamiliar device is identified, experts in CMR safety should be consulted prior to scanning. If the type of device cannot be determined, alternatives to CMR should be considered, and the potential risks of patient injury weighed against benefits before proceeding. In addition, inpatients should be examined prior to scanning for the presence of temporary devices such as catheters or temporary pacing leads. If an unreported metallic object is discovered during scanning, the scan should be stopped and the patient questioned further until the object is identified.
Safety Terminology and Labeling
Terminology regarding implants and devices in the MR environment [73] has evolved over time, as outlined in Table 8.2. In 1997, the FDA Center for Devices and Radiological Health (CDRH) proposed the terms “MR safe” and “MR compatible” (Table 8.2, Older terminology) for labeling MR information relating to medical devices [18]. Testing for MR safety involved assessing the effects on the device of the “MR environment”, including the static, time-varying gradient and RF electromagnetic fields produced by the MR system. Testing for MR compatibility involved safety testing plus characterization of any image artifacts produced by the device.
Table 8.2
Terminology used for labeling implanted devices
Older terminology | |
MR safea: | The device, when used in the MR environment, has been demonstrated to present no additional risk to the patient, but may affect the quality of the diagnostic information |
MR compatiblea: | The device, when used in the MR environment, is MR safe and has been demonstrated to neither significantly affect the quality of the diagnostic information nor have its operations affected by the MR device |
Newer terminology < div class='tao-gold-member'>
Only gold members can continue reading. Log In or Register a > to continue
![]() Stay updated, free articles. Join our Telegram channel![]() Full access? Get Clinical Tree![]() ![]() ![]() |