![]() Figure 32.1. Fast spin-echo axial image of a large atheromatous plaque protruding in the aortic lumen of the descending aorta (arrows). |
so that the images are reconstructed in the different phases of the cardiac cycle. Gradient-echo images are acquired with a high degree of temporal resolution throughout the cardiac cycle (8 to 16 frames per second) and can be displayed in cine format. Flow-related enhancement is produced by inflow of unsaturated blood exposed to only one RF pulse. As a result, the laminar-moving blood displays bright signal in contrast to stationary tissues. Signal can be reduced if the flow is low, as in aortic aneurysms. Mural thrombi can be identified by persistently low signal intensity in different phases of the cardiac cycle. Turbulent flow produces rapid spin dephasing and results in a signal void. This phenomenon makes it possible to detect anomalous turbulence, such as aortic or mitral insufficiency or jetlike communication between the true and the false lumen in aortic dissection. Despite the use of flow compensation, turbulent flow can be also observed in the normal aorta especially on the inner wall of the aortic arch. Recently, high performance gradient systems with even faster acquisition (TE, 2 to 3 milliseconds; TR, 4 to 8 milliseconds; flip angle, 20 degrees), have provided highquality images of the entire aorta at 15 to 25 different levels in less than 10 minutes. Gradient-echo images can provide additional information in many pathologic conditions such as coarctation, aortic valve insufficiency, and aortic aneurysm and dissection (14). Particularly, in aortic dissection the detection of entry and re-entry sites is a special capability of functional MRI that can be helpful in planning both surgical and endovascular therapy.
TABLE 32.1 General Strategy of MR Study of Thoracic Aorta | ||||||||||||||||||||||||||||||||||||||||||
---|---|---|---|---|---|---|---|---|---|---|---|---|---|---|---|---|---|---|---|---|---|---|---|---|---|---|---|---|---|---|---|---|---|---|---|---|---|---|---|---|---|---|
|
is saturated by the rapid repeated application of RF pulses. To provide a flow signal before phase dispersion, the TE is kept very short (<10 milliseconds) and the 180-degree refocusing pulse is eliminated. Fresh blood flowing into the plane of imaging produces a bright signal in a dark background. Venous signal, which can confuse the arterial image, can be eliminated with presaturation pulses. The use of a segmented gradient-echo sequence with cardiac triggering is helpful to eliminate arterial pulsation artifacts. TOF images can be acquired in two-dimensional (2D) or 3D fashion. Two-dimensional TOF imaging is very fast but poor in resolution, whereas high-resolution 3D images require 10 to 20 minutes of acquisition time. The advantages of both 2D and 3D techniques are gained with a series of thin-slab 3D acquisitions. The sequential 3D (multiple overlapping thinslab acquisition [MOTSA]) technique provides better flow enhancement than single-slab 3D techniques and causes less dephasing than 2D techniques. However, with sequential 2D or 3D acquisitions, even slight movement by the patient can generate discontinuities in the vessel contour.
Ultrafast 3D acquisition in conjunction with fast table feeds also makes it possible to chase the contrast bolus through several vascular districts. Since aortic aneurysm and dissection are mainly related to atherosclerotic vascular disease, a noninvasive concomitant assessment of the entire vascular system is advisable before surgical planning.
![]() Figure 32.2. Gadolinium-enhanced MRA in a patient with type A dissection (maximum intensity projection algorithm). The intimal flap is visible in both, the ascending and descending aorta (asterisk). |
TABLE 32.2 Example of SDCT and MDCT Scan Protocols in Aortic Evaluation | ||||||||||||||||||||||||||||||||||||||||||||||||||||||||||||||||||||||||||||||||
---|---|---|---|---|---|---|---|---|---|---|---|---|---|---|---|---|---|---|---|---|---|---|---|---|---|---|---|---|---|---|---|---|---|---|---|---|---|---|---|---|---|---|---|---|---|---|---|---|---|---|---|---|---|---|---|---|---|---|---|---|---|---|---|---|---|---|---|---|---|---|---|---|---|---|---|---|---|---|---|---|
|
enhancement has been reported using a standard (empirical) start delay (42), individual tailoring of the bolus injection is required in order to maximize arterial enhancement in differences of circulation time (43,44). This can be performed either by test bolus acquisitions or by bolus tracking algorithm sequences. The first technique requires an additional injection of a small contrast volume (10 to 20 mL) before the acquisition of CTA data, followed by the use of the same injection parameters. Bolus tracking modality permits the initial injection of the whole contrast agent volume, while the start of data acquisition is triggered by an automated detection of the contrast bolus arrival, using preconfigured trigger thresholds. An ROI is placed into the target vessel and the attenuation level (expressed in Hounsfield unit) is monitored within the ROI in real time during a single-level dynamic scan.
TABLE 32.3 SDCT and MDCT Contrast Injection Parameters in Study of the Aorta | ||||||||||||||||||||||||||||||||||||||||||||||||||||||||||||||||||||||||
---|---|---|---|---|---|---|---|---|---|---|---|---|---|---|---|---|---|---|---|---|---|---|---|---|---|---|---|---|---|---|---|---|---|---|---|---|---|---|---|---|---|---|---|---|---|---|---|---|---|---|---|---|---|---|---|---|---|---|---|---|---|---|---|---|---|---|---|---|---|---|---|---|
|
urgent surgical repair whereas most of type B dissections can be successfully managed with medical therapy.
slow flow from thrombus in the false lumen (56,57). However, because the diagnosis of aortic dissection is not dependent on functional gradient-echo images, these sequences should be reserved to clinically stable patients.
TABLE 32.4 MR Study Modality in Acute Aortic Syndromes | |||||||||||||||||||||||||||||||||
---|---|---|---|---|---|---|---|---|---|---|---|---|---|---|---|---|---|---|---|---|---|---|---|---|---|---|---|---|---|---|---|---|---|
|
![]() Figure 32.5. Spin-echo sagittal image of type A dissection: The intimal flap is visible as subtle linear image in the ascending and descending aorta. |
![]() Figure 32.6. Gradient-echo sagittal image of type B dissection: Flow turbulence (signal void, arrow) in the descending aorta indicates the entry site. |
![]() Figure 32.7. MRA 3D (A) and MIP (B) images of aortic dissection (abdominal aorta): Celiac, mesenteric, and left renal arteries originate from the anterior true lumen (arrows). |
ascending aorta, can influence specificity in an operatordependent manner. Since TEE information is limited to the thoracic aorta, sometimes with suboptimal display also of the aortic arch, a second imaging modality encompassing the entire aorta is advisable in stable patients.
![]() Figure 32.8. MRA 3D (A,C) and MIP (B,D) images of aortic dissections; the false lumen is patent in (A,B) and partially thrombosed in (C,D) (asterisk). |
toward MRI or TEE as the most sensitive tests for aortic dissection. Unfortunately, much of this opinion is based upon comparative studies where MRI or TEE is compared with relatively obsolete conventional CT or SDCT techniques (53,59,60,63,64 and 65) (Table 32.5). To date there have been no comparisons of multidetector CT to either MRI or TEE and is useless to consider these prior studies while CT, more than MRI or TEE, got substantial advances and features.
TABLE 32.5 Comparison between CT—Standard (S) or Helical (H)— and TEE, MR, and Angiography in Aortic Dissection | ||||||||||||||||||||||||||||||||||||||||||||||||||||||||||||||||||||||||||||||||||||
---|---|---|---|---|---|---|---|---|---|---|---|---|---|---|---|---|---|---|---|---|---|---|---|---|---|---|---|---|---|---|---|---|---|---|---|---|---|---|---|---|---|---|---|---|---|---|---|---|---|---|---|---|---|---|---|---|---|---|---|---|---|---|---|---|---|---|---|---|---|---|---|---|---|---|---|---|---|---|---|---|---|---|---|---|
|
dissection. These pitfalls are attributable to technical factors (improper timing or rate of contrast administration), streak artifacts (high-attenuation materials, high-contrast interfaces, and cardiac motion), periaortic structures (aortic arch branches, mediastinal veins, pericardial recess, thymus, atelectasis, and pleural thickening or effusion), aortic wall motion, aortic anomalies, and the aforementioned aortic aneurysm with thrombus (68,76). At present with an optimal scan protocol which requires a precontrast study, an ECG-gated arterial phase sequence and a delayed scan for slower blood flows, most of these artifacts/pitfalls are minimized.
following blunt chest trauma (78). As in aortic dissection arterial hypertension is the most frequent predisposing factor. Clinical signs and symptoms and the prognosis do not differ to classic aortic dissection, and IMH should be regarded as a variant of dissection with similar or more serious prognostic and therapeutic implications (79). Typical complications of dissection such as fluid extravasation with pericardial, pleural, and periaortic hematoma may occur in IMH as well. In a retrospective analysis of the Yale experience on 214 patients with acute aortic syndromes, Coady et al. (80) found 47.1% rate of aortic rupture for IMH, higher than that for classic type A (7.5%) or type B dissection (4.1%). However, after the acute phase, the evolution of IMH may be also favorable; in the series described by Yamada et al. (81), nine of ten survivors to initial presentation showed complete resolution of the aortic hematoma within 1 year.
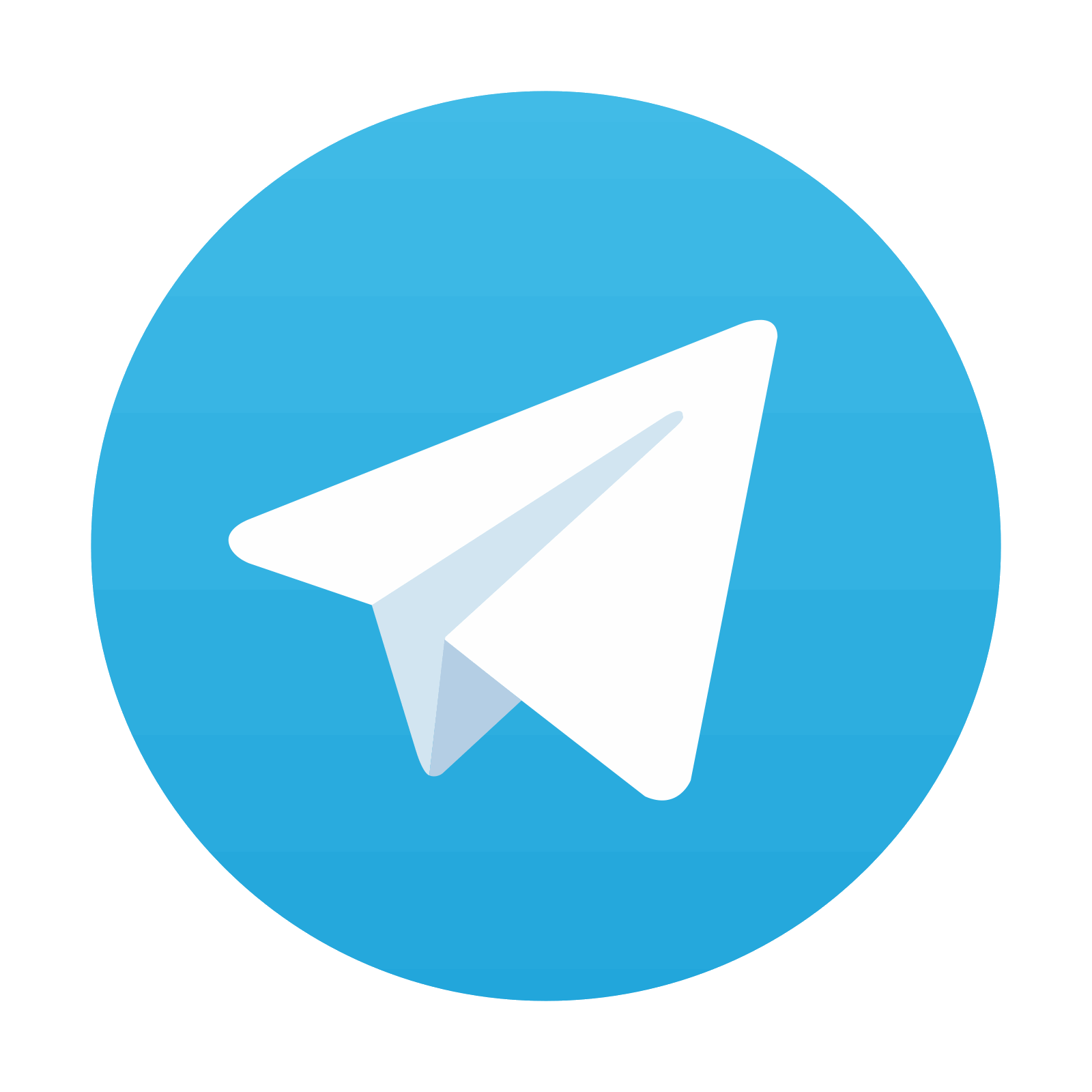
Stay updated, free articles. Join our Telegram channel
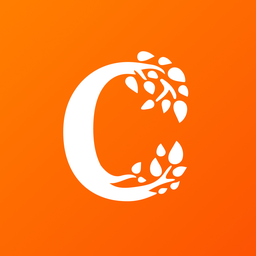
Full access? Get Clinical Tree
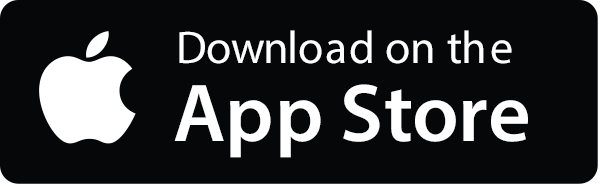
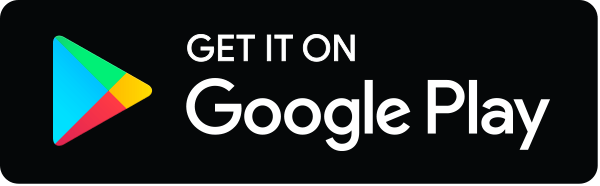