Fig. 2.1
Hematoxylin and Eosin stained, formalin-fixed lung sections from mice exposed by oropharyngeal aspiration 72 h previously to a phosphate-buffered saline or b 2.5 mg/kg of E. coli serotype 0111:B4 LPS. Note damage to the airway wall, thickening of alveolar septae, and inflammatory cell infilatrate, following LPS exposure. Ai—airway, Av—alveolus, V—vasculature. Note the alveolar septal thickening, alveolar inflammatory infiltrate, and airway wall injury in the LPS-exposed mouse lung
Bacterial Pneumonia Models
Bacterial infection is a commonly used and clinically relevant model of lung injury. In addition to modeling neutrophilic inflammation and alveolar capillary barrier dysfunction, use of live bacteria allows assessment of additional relevant host response questions, including bacterial clearance and bacterial dissemination. These additional parameters are particularly important when assessing potential new therapeutic interventions, given that pneumonia and sepsis are two of the most common causes of ARDS. Mice have varying susceptibility to different bacteria. C57BL/6 mice will clear Staphylococcus aureus and Pseudomonas aeruginosa without antibiotics and will survive infections with relatively high bacterial loads of 106–107 [36]. In contrast mice are highly susceptible to Klebsiella pneumonia, and intratracheal inoculation of 700 cfu in CBA/J mice results in ~20 % mortality by 72 h [37].
Bacteria can be aerosolized and delivered via a whole body or nose-only exposure system or given by oropharyngeal aspiration. The advantages of aerosolization include dosing a large number of mice simultaneously and achieving a uniform deposition in the lung, however, the cost for setting up an aerosolization system can be significantly higher. We use the AeroMP aerosol management platform combined with a whole body exposure chamber (Biaera Technologies, Hagerstown, MD), but other systems, including non-commercial set-ups are also effective. Additionally, when delivering bacteria by aerosol, preliminary experiments, in which mice are euthanized immediately after exposure for quantitative cultures of the lungs are necessary to define the relationship between aerosolization parameters (concentration, duration) and deposition.
The primary advantage of oropharyngeal aspiration is the ability to quickly get an infection model up and running without the need to optimize delivery parameters to achieve the desired inoculation. Additionally, higher loads of bacteria can be delivered by direct inoculation as opposed to aerosolization (Fig. 2.2). Finally, an argument can be made that oropharyngeal aspiration, which results in heterogeneous infection, better models clinical pneumonia. An example protocol for preparing S. aureus for infection is provided at the end of this chapter.


Fig. 2.2
Four mice imaged, using the IVIS in vivo imaging system 6 h after oropharyngeal administration of bioluminescent P. aeruginosa (Xen 05, Perkin Elmer). Mice are anesthetized with isoflurane and imaged in the supine posture. Note heterogeneous distribution typical of oropharyngeal aspiration in mouse 3 and mouse 4
Hyperoxia-Induced Lung Injury
Prolonged exposure to high oxygen fractions causes lung injury in a strain- and sex-dependent manner that is thought to be secondary to generation of reactive oxygen species, leading to peroxidation of membrane lipids, proteins, and nucleic acids and promotion of both necrotic and apoptotic cellular death [38]. In contrast to bacterial or LPS-induced lung injury, hyperoxia is associated primarily with disruption of the alveolar capillary barrier, and neutrophilic inflammation develops late in moribund mice. Exposure of female C57BL/6 mice to an inspired oxygen fraction of ≥0.95 results in measurable permeability changes by 36–48 h, neutrophilic emigration into the alveolar spaces by 72–84 h, and death by 96–120 h. In contrast, approximately 80 % of female C57BL/6 mice will survive exposure to an FiO2 of ~0.8 [39]. Although hyperoxia exposure systems for mice are commercially available, it is also relatively straightforward to fashion one. A non-airtight Plexiglas box, sufficient to hold one or more micro-isolator mouse cages and with a hinged end for access, is connected to an oxygen concentrator, capable of delivering oxygen at 5 lpm. A vacuum line is also attached to the box with vacuum sufficient to result in ~3 lpm air flow. As long as the box is not airtight, oxygen delivery in excess of the flow through the vacuum system will vent to the room and also result in an elevated oxygen fraction in the chamber. However, in the event of failure of the oxygen concentrator, the vacuum line will pull sufficient fresh ambient air through the exposure chamber to prevent build-up of CO2. Fine tuning of the actual oxygen concentration in the chamber can be achieved by adjusting the inspiratory oxygen flow and/or the vacuum flow.
Bleomycin-Induced Lung Injury
Bleomycin is an anti-neoplastic drug isolated from Streptomyces verticillus [40]. Intratracheal administration of bleomycin is typically thought of as a model of pulmonary fibrosis; however, in fact it causes injury that follows a well-defined pattern of acute neutrophilic inflammation and disruption of the alveolar capillary barrier that peaks by day 3–7 followed by resolution of inflammation and development of a fibroproliferative phase that peaks around day 21–24, which then resolves over time [41–43]. Dosing of bleomycin can be challenging; there is a narrow dose range in which significant lung injury develops, but the majority of animals recover. This is further complicated by a significant sex-dependent variation in susceptibility with female C57BL/6 mice being significantly more resistant to bleomycin-induced lung injury than male mice. A prudent practice is to prepare bleomycin in quantities sufficient to complete a series of experiments, aliquot and store at −20 ℃, and then perform an initial dose response experiment to determine the optimal dose for that particular preparation. Additionally, because of the potential concern for injury to the pharynx and larynx, most investigators will intubate mice and administer bleomycin intratracheally as opposed to administration by oropharyngeal aspiration. Several different approaches to orally intubating mice have been described [44–46].
Ventilator-Induced Lung Injury
Mechanical ventilation is commonly employed, clinically, to support patients with ARDS. However, beginning in the 1990s, clinicians recognized that mechanical ventilation can also worsen injury in patients with ARDS, culminating in a large, multi-center trial that demonstrated a large mortality benefit associated with reducing tidal volumes in patients with ARDS [47]. Additionally, retrospective studies have suggested that mechanical ventilation can increase the likelihood of ARDS developing in patients with ARDS risk factors [3, 4]. Similarly, mechanical ventilation in mice will induce lung injury as measured by histological changes, neutrophilic inflammation, and disruption of the alveolar capillary barrier. Mouse models of mechanical ventilation have used large tidal volumes and low or absent positive end-expiratory pressure (PEEP) in isolation to induce lung injury; however, this approach is limited by several factors, including relative resistance of mice to lung injury from ventilation alone, potential impact on hemodynamics, which are challenging to quantify and adjust in mice, and questions regarding the clinical relevance of such models. In contrast, mechanical ventilation with moderate tidal volumes in the range of 10–15 mL/kg synergistically increases lung injury when combined with bacterial products [26, 28, 48], infections [29], hyperoxia [32], and acid instillation [30]. The mechanisms by which mechanical ventilation interacts with other forms of lung injury to amplify the injury response are unclear and remains an active area of investigation. Mechanical ventilation, using parameters that do not result in overt lung injury, results in transcriptional activation of pathways associated with inflammation and may synergistically upregulate pro-inflammatory mediators associated with lung injury and development of extra-pulmonary organ dysfunction [29, 49–52]. Additionally, mechanical strain likely causes physical disruption of cellular membranes, particularly in lung epithelial cells [53, 54], resulting in release of damage-associated molecular patterns and inflammatory activation [28]. Mechanical ventilation in mice is technically challenging, particularly for periods longer than 4 h. A number of pitfalls can significantly increase mortality. For example, body temperature is difficult to regulate in a mouse that is anesthetized and supine, and typical warming pads placed under the mice are insufficient. Temperature regulation is better if the mouse is prone on a warming pad but limits access to the mouse. We have found that a heat lamp with a rheostat is the most effective method for maintaining body temperature of mechanically ventilated mice in the supine posture. However, body temperature must be monitored via a rectal thermistor or similar method to insure that it remains within an appropriate range. A second important issue is control of mouse respiratory effort. In our experience, total suppression of mouse respiratory effort is not feasible with anesthesia alone. Given that mouse ventilators generally are simple volume adjustable syringe pistons with a user set frequency, discordance between spontaneous respiratory effort and the ventilator’s respiratory cycle are inevitable. This is easily observed as variation in airway pressure over short intervals. To prevent spontaneous respiratory effort, neuromuscular paralysis with a non-depolarizing paralytic agent, such as pancuronium, vecuronium, or rocuronium is required. Such agents require a plan for monitoring adequacy of anesthesia, which is challenging in a paralyzed mouse. Our experience is that non-invasive blood pressure measurements are unreliable in anesthetized, mechanically ventilated mice. Invasive monitoring of blood pressure would be sufficient but is technically challenging and significantly increases preparation time and risk of unintended death. One option is to monitor heart rate via EKG and develop an algorithm with the Institutional Animal Care and Use Committee to identify parameters for anesthesia adjustment. For example, increases in heart rate of 20 % or more could trigger an increase in inhaled isoflurane concentration by 0.5 % or an additional dose of systemic anesthetic at half the original induction dose. Finally, monitoring for continued mouse viability is important. In our experience, anesthetized, paralyzed, ventilated mice can die on the ventilator without recognition by performing personnel. If airway pressure is monitored, then a rapid, large increase in airway pressure typically signals death. However, measurements that are more robust include monitoring exhaled CO2 or monitoring the EKG. A general description of a mouse mechanical ventilation setup is included at the end of this chapter.
Assessment of Lung Injury
A comprehensive workshop report by the American Thoracic Society has been published outlining assessment of lung injury in animal models and should be referred to for an in-depth discussion [7]. However, to summarize, the primary features of experimental acute lung injury in animals were identified as histological evidence of tissue injury, alteration of the alveolar capillary barrier, evidence of an inflammatory response, and evidence of physiological dysfunction. Of these parameters, quantitative assessment of alveolar capillary barrier and quantitative assessment of inflammation are the most commonly employed and easiest to measure. In our opinion, histological evidence of lung injury is most useful for a qualitative demonstration of injury and to identify potential anatomical localizations of specific processes, e.g., by IHC. This position paper proposed a semi-quantitative histology system that has been commonly employed; however, without a systematic sampling system such as designed-based stereology, the quantitative value of this approach is questionable [55].
Multiple approaches can be used to assess alveolar capillary barrier integrity, including assessment of extravascular fluid accumulation and permeability of the lung to macromolecules. The most common measure of extravascular fluid accumulation is wet lung weight at the time of necropsy, normalized either to the dry lung weight, obtained by drying the lung in an oven until weight no longer changes, or to pre-experiment total body weight. Disruption of the alveolar capillary barrier is commonly measured by presence of serum proteins in the alveolar compartment. The simplest, yet reliable, measure is to assay total protein in cell-free bronchoalveolar lavage fluid by the bicinchoninic acid or BCA assay. A brief protocol for mouse bronchoalveolar lavage is included at the end of this chapter. Another approach is to quantify IgM in bronchoalveolar lavage fluid, using a commercially available ELISA (e.g., Bethyl Laboratories). IgM exists in a pentameric form with a molecular weight of ~900 kD. It is not normally present in the alveolar compartment, and elevated levels imply frank disruption of the alveolar capillary barrier. Other techniques include injecting mice with Evan’s Blue dye at a dose of 20 mg/kg. Evan’s Blue dye labels albumin, in vivo, and translocates to the lung with albumin during lung injury. Permeability is then measured by flushing the pulmonary vasculature via the right ventricle, post-mortem, extracting the dye with formamide, quantifying by spectrophotometry [56]. An additional method is to intravenously inject mice with 100 µL of a 14.4 mM solution of a fluorescence-labeled 70 kD dextran (ThermoFisher Scientific) 1–3 h prior to euthanasia. Injections are most consistently accomplished via the retro orbital sinus in anesthetized mice. Degree of alveolar capillary permeability can be assessed by measuring the specific fluorescent label in bronchoalveolar fluid with a fluorimeter [28].
Assessment of cellular lung inflammation is easily assessed in bronchoalveolar lavage fluid. Because the number of cells present in the alveolar compartment of normal lungs are relatively low, counting can be facilitated by spinning out the cells from the lavage fluid and then suspending them in a smaller volume (0.2–0.5 mL). Total cell count is made using a hemocytometer, and percentage of neutrophils and mononuclear cells can be assessed on a cytospin preparation of 30,000 cells, using a modified Wright stain. Percentages of other cell types, such as lymphocytes and eosinophils are also often made; however, reliably distinguishing these specific cell types can be challenging and flow cytometry analysis should be considered. Release of pro-inflammatory cytokines can also be assessed via standard ELISA or multiplex assays on cell-free bronchoalveolar lavage fluid as well as tissue homogenate.
Assessment of physiological dysfunction is the most challenging lung injury parameter to obtain in mice. Measurement of gas exchange can be attempted with trans-cutaneous oximeters; however, these devices are frequently unreliable in anesthetized mice. We have found that the best reading is obtained on a mouse thigh after removing hair by a depilatory. Arterial blood gas measurements can also be attempted via left ventricular cardiac puncture. More recently, computer-controlled mechanical ventilators have been developed (e.g., flexiVent, SciReq), which allow application of defined forced oscillatory waveforms to mice. Measurement of pressure fluctuations with changing volume allows estimation of lung mechanics, including elastance and resistance [57]. These newer ventilators also allow measurement of more traditional pressure–volume curves for estimating lung compliance.
Many of these measures can be done in the same animal and a general guideline is that measuring lung injury by multiple different methods will increase confidence in the overall conclusions of the study. We routinely combine measurements of elastance over time, lung extravascular water as estimated by lung weight normalized to body weight, alveolar capillary disruption by measurement of total protein, IgM concentration, and extravasation of 70 kD fluorescence-labeled dextran, leukocyte total and subset counts in the bronchoalveolar lavage fluid, and assessment of pro-inflammatory cytokine release in bronchoalveolar lavage fluid.
Example Protocols
Oropharyngeal Aspiration (Fig. 2.3)
Materials needed


Fig. 2.3
Setup for oropharyngeal aspiration. a Equipment needed from left to right include an angled stand from which to suspend a mouse by its front incisors, a curved micro-forceps, a 200 μl pipet with aersol-resistant tips. b Anesthetized mice are suspended by their front incisors. The tongue is gently extended with the forceps, and 50 μl of instillate is introduced to the oropharynx. The tongue must be kept extended until the liquid is completely aspirated (~20 respiratory cycles)
Stand for suspending a mouse via front incisors (available commercially but a metal bookend, bent to a 75° angle with a rubber band stretched between two bolts works well)
Two small forceps
200 µL pipet
Anesthesia.
Oropharyngeal aspiration is a straightforward and relatively quick method to deliver material to the lungs. The primary limitation to this method is that distribution of material to the lungs is heterogeneous with a tendency toward greater delivery to the left lung. In a study comparing oropharyngeal aspiration to intratracheal instillation and nose-only aerosol delivery, the fractional distribution of a 99mtechnetium-labled sulfur colloid to the lungs by oropharyngeal aspiration was equivalent to that of intratracheal instillation and significantly higher than that observed with aerosolization [58]. Importantly, the optimal volume for aspiration in this study was identified as ~50 µL.
For oropharyngeal instillation, prepare the instillate at a concentration for which 50 µL or 2 µL/g body weight of the mouse will result in the desired dose. Load 50 µL or 2 µL/g body weight into a P200 pipet. Anesthetize the mouse. Although systemic anesthesia such as ketamine/xylazine will work, use of an inhaled anesthetic such as isoflurane in an exposure chamber provides adequate duration of anesthesia for the procedure and results in more rapid recovery of the mouse. The level of anesthesia should be sufficient that the mouse is unresponsive to handling, and respiratory rate has decreased and is notably deeper. At this level of anesthesia, the mouse is suspended by its front incisors from the stand. Using the two forceps, the oral cavity is exposed and the tongue is fully extended. This is a critical step as lack of tongue extension will result in swallowing of the instilled material as opposed to aspiration. The pressure exerted with the forceps must be sufficient to keep the tongue fully extended without causing significant tissue trauma, resulting in edema and impairment of subsequent food and water intake. Once the tongue is extended, the pipet tip is inserted into the posterior oropharynx and the liquid is instilled. At this point, the tongue must be kept extended until the liquid is fully aspirated as determined visually by clearance from the oropharyngeal cavity. Our practice is to typically keep the tongue extended for 5 breaths following clearance of the liquid from the oropharynx. Failure to keep the tongue extended during this period will result in variable amounts of the instillate being ingested as opposed to aspirated. Following instillation, the mouse is removed and recovered from anesthesia.
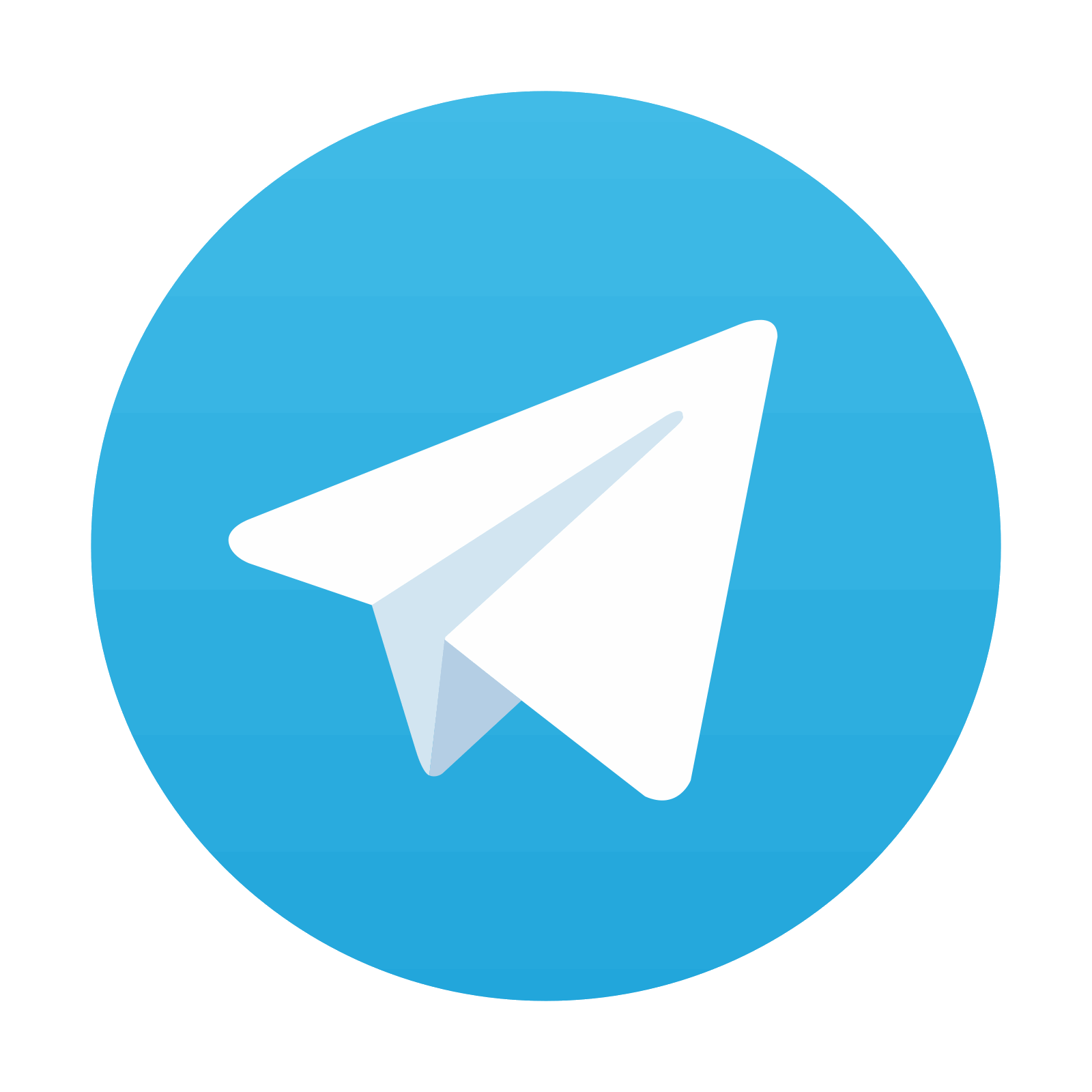
Stay updated, free articles. Join our Telegram channel
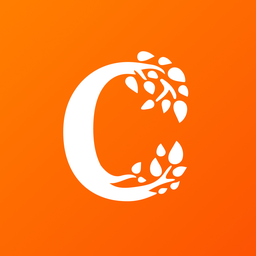
Full access? Get Clinical Tree
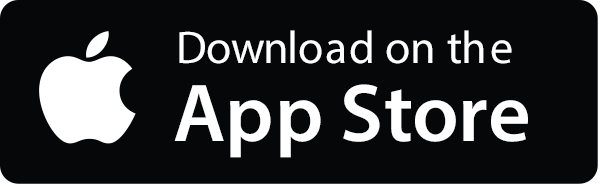
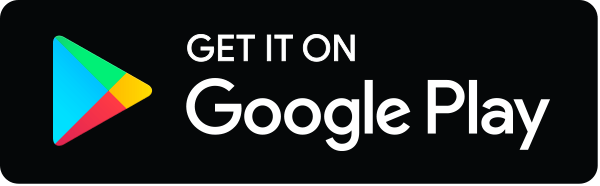