Abstract
Critically ill patients are vulnerable to organ injury and circulatory failure due to cellular hypoxia. Monitoring for perturbations in circulatory well-being, early recognition of tissue hypoxia, and prevention of organ injury via preservation of adequate oxygen delivery are at the core of critical care medicine. Oxygen must make the journey from the atmosphere to the intracellular environment to take part in the oxidative energy generation process. To sustain cellular obligatory and facultative functions, cells must consume adenosine triphosphate generated from oxygen. If oxygen delivery is limited, loss of facultative function occurs first and is then followed by loss of obligatory functions. Thus insufficient oxygen delivery creates a hypoxic tissue environment culminating in organ malfunction and followed by eventual organ death. This chapter provides a broad overview of the noninvasive and invasive monitoring modalities and biochemical markers commonly employed to assess the circulatory status and organ function in critically ill infants and children with acquired and congenital heart disease. Although each system is described in isolation, simultaneous use of multiple modalities in critical illness is necessary to quantify adequacy of oxygen delivery and to guide management during physiologic volatility. In fact, technologic innovation is dependent upon synchronized, concurrent integration of multiple systems and the electronic medical record for the development of advanced monitoring systems, real-time analytics, and risk prediction.
Key Words
monitoring, NIRS, CVP, pulse oximetry, capnography, mixed venous saturation
Critically ill patients are vulnerable to organ injury and circulatory failure due to cellular hypoxia. Monitoring for perturbations in circulatory well-being, early recognition of tissue hypoxia, and prevention of organ injury via preservation of adequate oxygen delivery are at the core of critical care medicine. Oxygen must make the journey from the atmosphere to the intracellular environment to take part in the oxidative energy generation process. To sustain cellular obligatory and facultative functions, cells must consume adenosine triphosphate (ATP) generated from oxygen. If oxygen delivery (DO 2 ) is limited, loss of facultative function occurs first and is then followed by loss of obligatory functions. Thus insufficient oxygen delivery creates a hypoxic tissue environment culminating in organ malfunction and followed by eventual organ death.
This chapter provides a broad overview of the noninvasive and invasive monitoring modalities and biochemical markers commonly employed to assess the circulatory status and organ function in critically ill infants and children with acquired and congenital heart disease. Although each system is described in isolation, simultaneous use of multiple modalities in critical illness is necessary to quantify adequacy of oxygen delivery and to guide management during physiologic volatility. In fact, technologic innovation is dependent upon synchronized, concurrent integration of multiple systems and the electronic medical record for the development of advanced monitoring systems, real-time analytics, and risk-prediction.
Noninvasive Monitoring
Electrocardiographic Monitoring
The electrocardiogram (ECG) is the graphic representation of the electrical activity of the heart, a necessary but insufficient component of myocardial function.
Since the introduction of continuous ECG monitoring more than 60 years ago, the goals of ECG monitoring in the cardiac intensive care unit (ICU) have expanded beyond simple heart rate and basic rhythm determination and currently encompass detection of complex arrhythmias, myocardial ischemia, conduction defects, and electrolyte abnormalities. Recent advances in rhythm monitoring systems expand beyond computerized arrhythmia analysis into advanced ST-segment ischemia analysis and predictive modeling. ECG algorithms are often limited by false alarms due to the need for high sensitivity at the expense of specificity. Hence human interpretation and oversight of ECG systems are still necessary. With these challenges in mind, it is essential that providers have a complete understanding of ECG monitoring systems, including their benefits and pitfalls.
The ECG is recorded by placing an array of monitoring electrodes in specific locations on the body surface to capture various axes of electrical vectors. The most commonly recognized ECG setup consists of a 12-lead system. Standard 12-lead ECG requires placement of electrodes on both the limbs and precordial position. Because this is difficult to achieve in a patient following cardiac surgery, simplified configurations are often used. They allow for limb lead placement on the torso to reduce muscle artifacts during limb movement and avoid lead cable tethering.
The basic ECG monitoring setup involves a three-electrode lead monitoring system ( Fig. 22.1A ). The potential difference between two electrodes is recorded and allows for monitoring of three leads (leads I, II, and III). The three leads record positive P and T waves as well as major portions of QRS complex. Bipolar lead monitoring provides a single-lead display and is often used for portable monitor-defibrillators because the system allows for evaluation of the heart rate and time relation between different waves of the cardiac cycle, detection of R waves for synchronized cardioversion, and detection of ventricular fibrillation. This type of monitoring is inadequate for the sophisticated arrhythmia and ST-segment monitoring required in the cardiac ICU because it lacks the anterior lead necessary to delineate arrhythmia origin.


The five-electrode lead system remains the most commonly used setup in the ICU and enables seven-lead monitoring (see Fig. 22.1B ). The leads from the extremities act as common ground (negative electrode) for the precordial unipolar lead (positive electrode), enabling display of leads I, II, III, aVR, aVL, aVF, and a single precordial lead (V 1 to V 6 ). The advantages of seven-lead monitoring are the ability to detect ischemic events utilizing ST-segment trending and to define the mechanism of arrhythmias as well as supraventricular tachycardia (SVT). It should be noted that although the V 1 lead location is excellent for diagnosis of wide QRS complex arrhythmias (bundle branch block, wide QRS tachycardia, and pacemaker rhythms), it is poorly sensitive in detection of myocardial ischemia. Leads III and V 5 appear to be the most sensitive pair for recognition of myocardial ischemia in the postoperative setting. More advanced 12-lead ECG systems are less frequently used in children compared with adults due to body surface limitation. If advanced arrhythmia or ischemia workup is required, the standard portable 12-lead ECG system should be employed.
All patients admitted to the ICU should be continuously monitored using a central telemetry system that records and stores ECG information, allowing for retrospective analysis. The standard for ECG recording is a 1-mV deflection equaling 10 mm on the strip chart recorder and with the paper speed set to 25 mm/s.
Arrhythmias are commonly encountered in the cardiac critical care unit with prevalence reported at 15% to 29%. The incidence of arrhythmias in the postoperative period for cardiac congenital repairs can be as high as 48%. Because postoperative arrhythmias are associated with significant morbidity and higher mortality, prompt diagnosis and appropriate management are crucial. Postoperative arrhythmias occur due to a variety of factors such as mechanical irritation of the conduction system, postoperative metabolic derangements, electrolyte disturbances, increased adrenergic tone from surgical stress, and use of inotropic medications. Surgical repairs involving ventriculotomies and coronary reimplantations predispose these children to transient ventricular dysrhythmias. Ventricular arrhythmias that occur in the setting of myocardial ischemia are associated with mortality as high as 61%. More common postoperative arrhythmias in children include junctional ectopic tachycardia (JET), SVT, and atrioventricular (AV) block, which might be difficult to diagnose using surface ECG.
Transcutaneous epicardial atrial leads ( Fig. 22.2 ), which record electrical activity directly from the atria and allow for pacing of atria and/or ventricles, can aid in diagnosis and management of complex rhythm and conduction disorders following open-heart surgery. P waves on the surface ECG may be buried in the QRS complex or T wave, complicating diagnosis of supraventricular arrhythmias such as JET. Atrial ECGs provide the ability to clearly identify atrial activity and localize P waves.

Myocardial ischemia in children in the postoperative setting can be the result of intraoperative hypoxia or mechanical stress of the coronary arteries due to prolonged cardiac surgery. Other described causes include acute myocarditis, postoperative pericarditis, acute graft rejection, transplant-related coronary artery disease, and cardiotoxicity from pharmacologic therapies and chest trauma. The ability to detect these complications via advanced ST-segment monitoring is uncertain. The ST amplitude is normally measured at a fixed interval of approximately 60 milliseconds after the J point. The steep slope of these ST segments cause ST amplitude to vary with heart rate triggering ST segment, resulting in frequent false ST alarms. For example, the presence of bundle branch block results in ST-T waves that markedly deviate in a positive or negative direction depending on the lead being monitored. Any questionable ECG changes especially those complemented by change in clinical status should always be evaluated with 12 lead ECG and additional diagnostic workup.
Software used for ST segment monitoring has undergone modifications to include trend analysis and noise reduction. This allows for improved ECG signal processing and measurement of ST-segment changes; however, little information is available regarding optimal lead systems for ischemia detection in children. When compared with Holter monitoring for ischemia in adults, the sensitivity and specificity of ST-trend monitors are described at 74% and 73%, respectively. The normal ST segment in a child is isoelectric with baseline elevations of the ST segment ±1 mm in the limb leads and up to ±2 mm in the precordial leads, but ST-segment elevations greater than 2 mm might be valuable in making the diagnosis of ischemia in children.
Pulse Oximetry
Pulse oximetry is a noninvasive spectroscopic technique that estimates arterial oxygen saturation. Since 1986 the American Society of Anesthesiologists has included in its “Standards for Basic Anesthetic Monitoring” that “during all anesthetics, a quantitative method of assessing oxygenation such as pulse oximetry shall be employed.” The American Academy of Pediatrics mandates the use of pulse oximetry for sedation in children. The widespread adoption of pulse oximetry as a safety monitor is intuitive, but evidence for such efficacy is surprisingly lacking. The widespread adoption of pulse oximetry to evaluate and monitor sick children has virtually eliminated unrecognized arterial hypoxia as a cause for patient harm, and it allows continuous assessment of right-to-left shunting in children with congenital heart disease.
Most modern pulse oximeters merge two physical principles: spectrophotometry and optical plethysmography. The spectrophotometry component of pulse oximetry operates on the theory that oxygenated hemoglobin (O 2 Hb) and deoxygenated hemoglobin (HHb) show different absorption in red (660 nm) and infrared light (940 nm). O 2 Hb absorbs more infrared light, whereas HHb absorbs more red light. The Beer-Lambert Law ( Eqs. 22.1a and 22.1b ) defines the attenuation of light through the material traveled:
T = I / I O
A = − log ( T ) = − log ( I / I 0 ) = ε ∗ b ∗ c
During the cardiac cycle the proportion of the light that strikes fat, connective tissue, skin, bone, and venous blood remains relatively constant (direct current). During systole a small increase in arterial blood volume increases light absorption (alternating current). Hence the total absorption (A total ) is time dependent (dt) during the cardiac cycle ( Eq. 22.2 ) with more absorption during arterial dilation (A arterial )—systole—and less during arterial constriction (A venous )—diastole ( Fig. 22.3 ).
d ( A total ) / d t = d ( A arterial ) / d t + d ( A venous ) / d t = d ( ε arterial ∗ b arterial ∗ c arterial ) / d t + d ( ε venous ∗ b venous ∗ c venous ) / d t

Under the assumption that arterial pulsation is much more than venous pulsation during the cardiac cycle (b venous is constant), the total absorption over time is almost exclusively dependent on the arterial absorption.
d ( A total ) / d t = d ( A arterial ) / d t = Δ A total = Δ A arterial
By comparing the peak absorption during arterial pulsation (alternating current) to a baseline, through absorption (direct current), only the arterial pulsation remains relevant ( Eq. 22.4 ) :
A = A C / D C
The combination of the ratios of absorbances at two distinct wavelengths (660 nm and 940 nm) with distinct absorbance characteristics for O 2 Hb and HHb ( Fig. 22.4 ) leads to a ratio of ratios ( Eq. 22.5 ):
R = A 660 nm / A 940 nm = ( A C 660 nm / D C 660 nm ) / ( A C 940 nm / D C 940 nm )

Averaged over a series of measurements, R is expressed on a calibration curve based on healthy volunteers with alterations of their saturations from 70% to 100%, where an almost linear relationship from R to saturations is defined ( Fig. 22.5 ).

The pulse oximeter with two wavelength measurements can distinguish a maximum of two substances: O 2 Hb and HHb. Hence pulse oximeter measurements are referred as functional saturations, defined as the ratio of O 2 Hb compared to total hemoglobin ( Eq. 22.6 ):
Functional SpO 2 = O 2 Hb / ( O 2 Hb + HHb )
Pulse oximeters are empirically calibrated on normal, healthy volunteers during desaturation studies. The Food and Drug Administration and US Department of Health and Human Services mandates an accuracy between oxygen saturation as measured by pulse oximetry (SpO 2 ) and measured arterial oxygenation by A rms ≤ 3.5% under normal conditions with SpO 2 ranging from 70% to 100%. A rms is defined as the root mean square, which reports accuracy as a function of bias and precision. All modern pulse oximeters meet those requirements under normal conditions; however, there are significant limitations in bias and precision of pulse oximeter readings in various settings ( Table 22.1 ).
Hypoxia and congenital heart disease | Pulsoximetric measured saturations below 70% show an increased bias and decreased precision. Multiple studies confirmed that the assessment of hypoxic states is unreliable and should be reconfirmed by cooximetric assessment. |
Hyperoxia | Hyperoxia (cooximetric measured saturations >100 mm Hg or >150 mm Hg) may lead to increased mortality in critical care patients. SpO 2 measurements are not reliable to detect hyperoxic states. |
Increased carboxyhemoglobin (COHb) | Significant COHb poisoning increases false high readings of SpO 2 ; recent advances in pulse oximeters use multiple wavelengths to quantify COHb and MetHb. |
Increased methemoglobin (MetHb) | MetHb absorbs light at almost similar wavelengths (660 nm/940 nm) as conventional pulse oximeter readings; hence in the presence of MetHb, SpO 2 readings are commonly between 82% and 86%; recent advances in pulse oximeters use multiple wavelengths to quantify COHb and MetHb. |
Hemoglobinopathies a | Hemoglobin Sydney and hemoglobin Southampton lead to falsely low SpO 2 . |
Body temperature | Increased body temperature causes more venous pulsation, which results in lower SpO 2 readings. |
Cardiac arrhythmia | See reference . |
Poor perfusion | See reference . |
Excessive ambient light | See reference . |
Motion artifacts | See reference . |
Probe miscalibration | |
Probe misplacement | |
Nail polish | Although traditional pulse oximeters may have been susceptible to nail polish decreasing SpO 2 reliability, newer models yield almost no difference in healthy volunteers. |
a The absorbance of red and infrared light by fetal hemoglobin (HbF) is essentially the same as adult hemoglobin (HbA), and thus SpO 2 measurement is as reliable in newborns as in adults.
The application of the pulse oximeter reaches far beyond its initial application for measurement of functional saturation and heart rate. During systole AC increases and more light is absorbed, whereas in diastole only DC contributes to the light absorption. The alteration of AC and DC during the cardiac cycle is a mirror image of an arterial blood pressure waveform, which is often displayed as a plethysmographic waveform. Further parameters can be derived as displayed in Table 22.2 .
Perfusion Index (PI) | PI = AC/DC * 100% | PI reflects the peripheral vasomotor tone. Low PI suggests peripheral vasoconstriction (or severe hypovolemia), and high PI suggests vasodilation. |
Pleth Variability Index (PVI) | PVI = (PImax − PImin) / PImax * 100% | PVI quantifies the variability due to respiration and is thought to be a surrogate measure of intravascular volume. |
Pulse oximetry is one of the standard tools for patient monitoring during anesthesia, the critical care setting, and even the acute care setting. Aside from the original application of the pulse oximeter for noninvasive arterial saturation and heart rate monitoring, recent improvements enable the use of the pulse oximeter in more challenging situations with low perfusion or hypoxemia, and analysis of the plethysmographic waveform may lead to more clinical information in the future.
Near-Infrared Spectroscopy
Optimal oxygen delivery to tissue beds occurs through not only global, but regional circulatory mechanisms. Regional perfusion is determined by perfusion pressure and regional vascular resistance, affected by local, humoral, and neural mechanisms that have complex inputs. Near-infrared spectroscopy (NIRS) provides a continuous, noninvasive estimate of regional tissue oxygen saturation that is highly venous weighted and thus can be conceptualized as an estimate of regional venous oxygen saturation, indicating changes in regional oxygen economy that occur both in normal circulatory adaptations and in more serious circulatory disturbances, including developing shock states. NIRS assists in the characterization of regional and global circulatory function directly and by facilitation of continuous, noninvasive application of the Fick principle.
NIRS utilizes a modified Beer-Lambert principle to provide a continuous noninvasive assessment of tissue oxygenation through quantitative assessment of the color of hemoglobin in blood in the optical field beneath the probe. Because approximately two-thirds of the total blood volume resides in the venous circulation, the optical field coverage is predominantly a venous-weighted tissue hemoglobin-oxygen saturation (rSO 2 ) and thus provides an estimation of the regional oxygen supply-demand relationship. Continuous, real-time changes in rSO 2 reflect changes either in metabolic demand or supply: as an approximation of regional venous oxygen saturation (SvO 2 ), rSO 2 = SaO 2 − VO 2 /DO 2 (where SaO 2 is arterial oxygen saturation and VO 2 is oxygen consumption) and is thus a function of systemic cardiac output (CO), arterial oxygen content, and total or regional resistance. Clinical studies have initially focused on cerebral NIRS and have validated cerebral rSO 2 with jugular bulb venous saturation (SjvO 2 ) measurements. The strongest correlation between rSO 2 and SjvO 2 has been observed in infants less than 10 kg (r = 0.83).
Several studies have reported the relationship between cerebral rSO 2 , somatic rSO 2 , and venous saturation from the superior vena cava (SvO 2 ). Because the mixed venous saturation is the flow-weighted average of regional venous saturations, attempts to show a univariate correlation between single-site rSO 2 and a mixed venous measure should be viewed as oversimplified. A global, quasi-mixed SvO 2 can be reasonably approximated by NIRS models that include multiple sites ( Fig. 22.6 ).

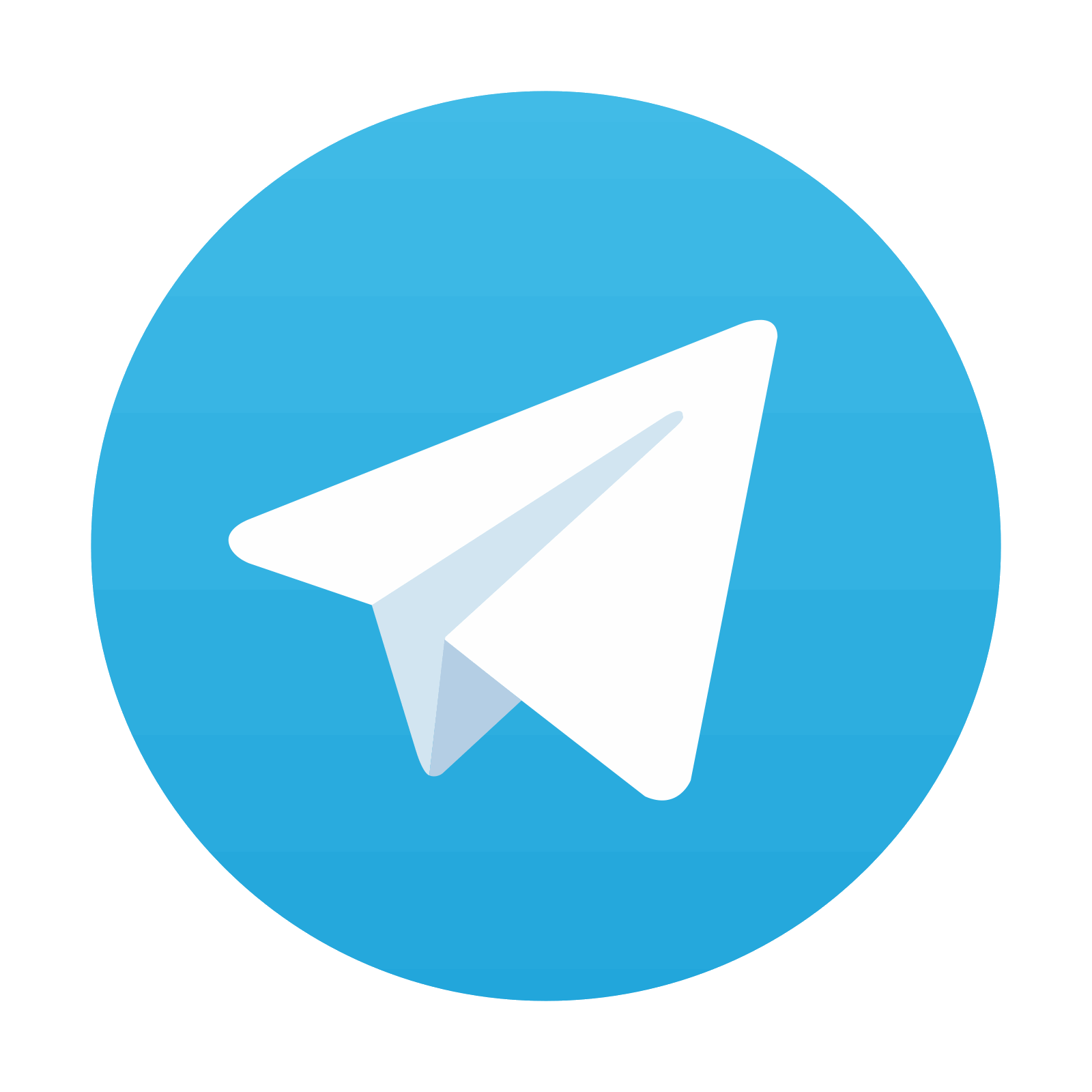
Stay updated, free articles. Join our Telegram channel
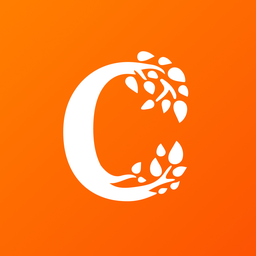
Full access? Get Clinical Tree
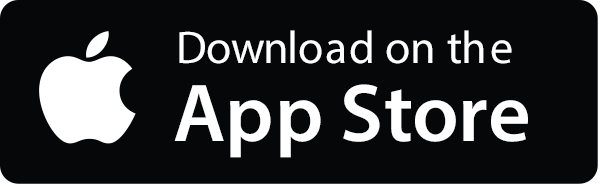
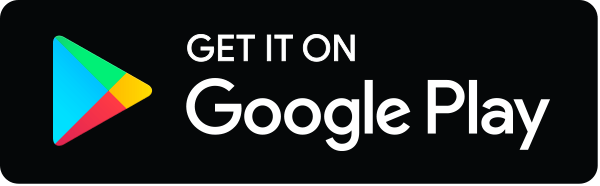
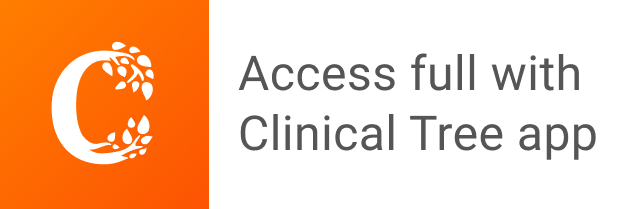