Fig. 18.1
Calcineurin/NFAT signaling plays a central role in the development of pathological cardiac hypertrophy. This pathway is an excellent example to illustrate how numerous molecules can act as modifiers and affect calcineurin/NFAT activity. These modifiers represent interesting targets in the treatment of cardiac hypertrophy
18.2.3 Histone Deacetylases (HDAC)
Lysine-(K)-deacetylases (KDACs) are important tools in eukaryotic posttranslational regulation as these enzymes control protein function by removing acetyl groups from modified lysine residues in a variety of nuclear and cytoplasmic target proteins [82]. Because lysine deacetylation was initially identified [83] and is best characterized in histones, eukaryotic KDACs are often referred to as histone deacetylases (HDACs). By removing acetyl groups from the N-terminal tails of histones and interacting with other chromatin regulators, HDACs promote nucleosome formation and chromatin condensation, which usually results in transcriptional repression of the targeted chromosomal region [84, 85]. Aside from gene regulation, HDACs have been shown to be associated with a multitude of other cellular processes, as they can as well act on nonhistone substrates, like transcription factors, signal transducers, hormone receptors, cytoskeletal proteins, and chaperones [82, 86].
Currently, 18 different and distinctly encoded HDACs have been identified in mammalian cells. These are divided into four classes: class I (HDACs 1, 2, 3, 8), subclass IIa (HDACs 4, 5, 7, 9), subclass IIb (HDACs 6, 10), class III (sirtuins), and class IV (HDAC 11) [87]. While class III HDACs require the NAD+ cofactor for activity, HDACs from the classes I, II, and IV are dependent upon Zn2+ for deacetylase activity.
Due to the fact that many redundant pathways converge on HDACs and their role as epigenetic regulators of gene expression, these enzymes represent interesting targets for therapeutic strategies. Especially since it was discovered that HDACs play an important role in cancer development and maintenance, several small-molecule HDAC inhibitors have been developed to counter tumor growth and tumor survival [88]. The first HDAC inhibitor approved by the FDA in 2006 for the treatment of cutaneous T-cell lymphoma was a pan-inhibitor of zinc-dependent HDACs called SAHA (vorinostat) [89]. When it became apparent that class IIa HDACs interact with the myocyte enhancer factor-2 (MEF2) transcription factor family and subsequently influence calcium/calmodulin-dependent protein kinase (CaMK)-and mitogen-activated protein kinase (MAPK)-mediated signaling [90], several studies focused on the role of HDACs in the heart.
The cardiac-specific transcription factor MEF2 is known to be a common endpoint of various hypertrophic signaling pathways. Interestingly, the posttranslational (in)activity of MEF2 is at least in part dependent upon its association with class IIa HDACs, which function as nuclear repressors of the transcription factor and silence the expression of MEF2 target genes [91, 92]. Consequently, it was shown that the overexpression or constant activation through signal-resistant mutants of class IIa HDACs 4 [93], 5 [94, 95], and 9 [95] suppresses MEF2-dependent gene expression and agonist-induced hypertrophic growth in primary cardiomyocytes. Consistently, the targeted deletion of HDAC 5 [96] or HDAC 9 [95] in mice results in spontaneous hypertrophy with advanced age. Furthermore, these knockout mice are sensitized to cardiac hypertrophy due to pressure overload. Additionally, transgenic mice displaying a moderate cardiac-specific overexpression of the class III HDAC Sirt1 show resistance to oxidative stress and apoptosis [97], whereas the chemical inhibition of Sirt1 in cardiomyocytes enhances apoptosis [98]. However, high-level overexpression of Sirt1 causes hypertrophy, which might be a result of mitochondrial dysfunction. Mice deficient for Sirt7 develop hypertrophy with extensive fibrosis and inflammatory cardiomyopathy [99]. Therefore, class IIa and III HDACs are generally seen as cardiac protectors.
In class I HDACs, redundancy of the different family members leads to conflicting assumptions of their function in the heart. In mice, the simultaneous cardiac-specific deletion of HDAC1 and HDAC2 results in neonatal lethality that is characterized by arrhythmias, dilated cardiomyopathy, and heart failure, whereas the cardiac-specific inactivation of either HDAC1 or HDAC2 has no apparent effect on the phenotype [100]. Inconsistently, different groups demonstrated that the knockout of HDAC2 is either insufficient to block hypertrophy in response to chronic administration of the β-adrenergic receptor agonist isoproterenol and pressure overload [100] or blunt the hypertrophic response to these stimuli [101]. On the other hand, transgenic mice that are overexpressing HDAC2 in the heart show severe cardiac hypertrophy [101]. Interestingly, heart-specific overexpression of HDAC3 results in increased cardiomyocyte proliferation and cardiac hyperplasia without hypertrophy [102]. The isoproterenol-induced hypertrophy was neither exacerbated nor inhibited by overexpression of HDAC3 in vivo.
As the class I HDACs seem especially detrimental to cardiac function, the emerging HDAC inhibitors represent potentially important tools in the treatment of cardiomyopathy. HDAC inhibitors are divided into four structurally distinctive groups, hydroxamic acids (e.g., trichostatin A [TSA], suberoylanilide hydroxamic acid [SAHA]/vorinostat), benzamides (e.g., MS-275), short-chain fatty acids (e.g., phenylbutyrate, valproic acid), and cyclic peptides (e.g., depsipeptides). The selectivity for different HDACs and the inhibitory potentials differ greatly among and within these groups [103]. Indeed, it was demonstrated that in mice, TSA attenuates ventricular hypertrophy that is induced by continuous infusion of isoproterenol [104], angiotensin II [105], or aortic banding [105, 106]. Of even greater significance is the fact that TSA is able to cause regression of a preestablished aortic constriction-induced cardiac hypertrophy [105]. Similarly, the hydroxamic acid scriptaid is able to reduce hypertrophy in the aortic banding model, which is accompanied by reduced cardiomyocyte size and preservation of systolic function [106]. Furthermore, both TSA and valproic acid blunted the development of cardiac hypertrophy in transgenic mice overexpressing the HDAC2-dependent SRF inhibitor Hop [104]. Of note, some of the observed effects could be due to a role of HDACs in the regulation of blood pressure, as valproic acid was, e.g., shown to affect the mean arterial pressure in spontaneously hypertensive rats [107].
HDAC inhibitors offer a novel therapeutic strategy to counter pathological cardiac hypertrophy and remodeling. Although HDAC inhibitors such as vorinostat or romidepsin are already approved by the FDA and are well tolerated in cancer treatment, little is known about long-term side effects. Interestingly, SAHA was recently tested in a mouse and in a rabbit model of ischemia/reperfusion [108]. In rabbits, treatment with SAHA before ischemia and at the time of reperfusion decreased infarction size and partially rescued cardiac function. Of note, SAHA seems to mediate its cardioprotective effects via an increased autophagic flux.
18.2.4 Small G Proteins
The family of small G proteins consists of numerous members that can be classified as Ras, Rho, Arf, Ran, Rab, and other proteins [109]. All of these proteins are monomeric GTPases that can either exist in an active form if bound to GTP or in an inactive form if bound to GDP. The switch between these two states is regulated by guanine dissociation inhibitors (GDI) and guanine nucleotide exchange factors (GEF). Furthermore, the GTPase activity can be enhanced by GTPase-activating proteins (GAP) [110].
18.2.4.1 Ras
Harvey-Ras (H-Ras), Kirsten-Ras (K-Ras), and neuroblastoma-Ras (N-Ras) are classical members of the Ras (rat sarcoma virus) family. Active Ras initiates MAPK signaling via Raf1, MEK1/MEK2, and ERK1/ERK2 (reviewed in [111]). In humans, mutations in genes of this particular pathway cause congenital disorders such as Costello, LEOPARD, and Noonan syndrome that are associated with hypertrophic cardiomyopathy [112]. Moreover, overexpression of Ras in the heart results in cardiac hypertrophy with myofibrillar disarray and diastolic dysfunction [113]. Consistently, mice that are deficient for the cardiac GAP Nf1 develop hypertrophy due to sustained activity of Ras finally resulting in heart failure and increased mortality [114]. Accordingly, cardiac-specific overexpression of a dominant negative isoform of Raf1 prevented transgenic mice from MAPK activation and hypertrophy after aortic banding [115]. However, transgenic animals showed increased mortality following pressure overload probably due to increased apoptosis of cardiomyocytes. In line with this notion, the cardiac-specific knockout of Raf1 was associated with an increase of apoptosis as well. These mice developed cardiac dysfunction and dilation but no hypertrophy [116]. Interestingly, elevated phosphorylation levels of p38 and JNK could be detected in the mutant hearts indicating activation of important hypertrophic pathways that are discussed elsewhere [117].
18.2.4.2 Rho
The small G protein RhoA induces ANP and MLC-2 expression as well as increased myofibrillar organization in cardiomyocytes in vitro [118, 119]. Although the overexpression of RhoA produces characteristic features of cardiomyocyte hypertrophy in cell culture, the cardiac-specific overexpression of RhoA did not result in a hypertrophic phenotype in vivo. Transgenic mice that overexpressed either a wild-type or a constitutively active form of RhoA did not develop cardiac hypertrophy [120], but left ventricular dilation and heart failure. Interestingly, these animals also exhibited bradycardia, atrioventricular blocks, and atrial fibrillation. The Rho-associated kinases ROCK1 and ROCK2 are effectors of RhoA. The homozygous deletion of ROCK1 in mice resulted in embryonic or postnatal lethality in most of the mice [121]. The heterozygous animals showed a significant reduction of ROCK1 and were analyzed after hypertrophic stimulation by chronic angiotensin II infusion and aortic banding. The hypertrophic response did not differ between wild-type and knockout mice. However, mutant animals developed less fibrosis. A ROCK1 knockout mouse model generated by a different group presented a similar phenotype. The analysis of homozygous ROCK1-deficient animals produced comparable results with less fibrosis upon transverse aortic constriction but no changes in cardiac hypertrophy. Consistently, the cardiac-specific overexpression of a constitutively active ROCK1 that lacks the inhibitory C-terminus caused fibrosis without hypertrophy [122]. The extent of fibrosis was exaggerated after treatment with angiotensin II compared to control mice.
The examples of the small G protein family outlined above emphasize the complexity of hypertrophic signaling. Still, little is known about the regulation and the effectors of these GTPases as well as potential targets for therapy.
18.2.5 Wnt/Frizzled Signaling
Frizzled receptors belong to the family of GPCRs that are discussed above. The function of Frizzled receptors however does not solely depend on the action of G proteins. Wnt proteins serve as ligands to activate Frizzled. Downstream intracellular signaling is mediated either via β-catenin, the so-called canonical pathway, or via noncanonical pathways [123]. In canonical signaling, Wnt stimulation results in recruitment of the coreceptors LRP5 and LRP6 to Frizzled, translocation of Axin and Dishevelled-1 to the receptor complex at the plasma membrane, and finally release of β-catenin from the destruction complex and translocation to the nucleus where it induces Wnt-dependent gene expression together with the transcription factors TCF/Lef (T-cell factor/lymphoid enhancer factor).
Without binding of Wnt ligands to Frizzled, β-catenin is incorporated into a destruction complex consisting of Axin, APC (adenomatous polyposis), GSK3β, and CK1 (casein kinase 1). CK1 acts as a priming kinase and phosphorylates β-catenin followed by phosphorylation of β-catenin by GSK3β. Phosphorylated β-catenin is ubiquitinated by the E3 ligase β-TrCP (β-transducin repeat-containing protein) and then degraded in the proteasome. In the absence of β-catenin, TCF/Lef is bound to transcriptional repressors of the HDAC family and Groucho [124].
The noncanonical pathways include activation of Rho/ROCK or Rac/JNK via Dishevelled-1 and phospholipase C via G proteins finally resulting in increased calcineurin and protein kinase C activity [124].
Since β-catenin serves as an integrator of canonical Wnt signaling, its role in cardiac hypertrophy is of special interest. Stimulation of cardiomyocytes with phenylephrine and endothelin-1 leads to accumulation of β-catenin. Furthermore, β-catenin levels increase after aortic constriction in rats [125]. The overexpression of a β-catenin mutant that lacks phosphorylation sites for GSK3β in cardiomyocytes causes hypertrophy [125]. The deletion of β-catenin in a constitutive knockout mouse model was lethal during embryogenesis [126]. Similarly, the heart-specific loss of β-catenin was embryonically lethal as well [127]. Heterozygous mice survived though and developed less cardiac hypertrophy following aortic banding [127]. Consistently, an inducible cardiomyocyte-specific knockout caused reduced hypertrophy due to pressure overload in mice [128]. Of note, analysis of the hypertrophic gene program revealed that the attenuated hypertrophic response was not associated with reduction of ANP and BNP expression in either of these models. Another model of inducible β-catenin deletion in the heart however did not alter hypertrophy induced by angiotensin II infusion [129]. Moreover, mutant mice that express a stabilized β-catenin by deletion of exon 3, which protects β-catenin from degradation, did neither develop hypertrophy under baseline conditions nor under stimulation with angiotensin II [129].
Experimental modulation of Wnt signaling upstream of β-catenin includes the deletion of Dishevelled-1 in mice [130]. Dishevelled-1 knockout mice exhibited blunted hypertrophy after aortic banding accompanied by reduced β-catenin levels. In line with these results, cardiac-specific overexpression of Dishevelled-1 in transgenic mice caused hypertrophy, cardiac dysfunction, and increased mortality [131].
Due to the partially contradictory studies analyzing the role of β-catenin in cardiac hypertrophy, the therapeutic exploitation of canonical Wnt signaling seems to be out of reach presently. Though, exploration of regulatory proteins that act upstream of β-catenin such as secreted Frizzled-related protein (sFRP), Dickkopf (DKK), and Wnt inhibitory factor 1 (WIF-1) might be useful. In line with this notion, it has been shown that DKK3 knockout mice are sensitized to pressure overload. These animals developed exaggerated hypertrophy following aortic constriction, while transgenic mice that overexpress DKK3 were protected after aortic banding [132].
18.3 Other Signaling Targets
18.3.1 Autophagy
Autophagy is a lysosome-dependent degradation mechanism that is responsible for recycling of intracellular components ranging from long-lived proteins to organelles such as mitochondria [133]. There are three major types of autophagy: macroautophagy, microautophagy, and chaperone-mediated autophagy [134]. Macroautophagy describes the event of engulfment of cytosolic material into emerging double-membrane vesicles, the so-called phagophores. The complete vesicles termed autophagosomes finally fuse with lysosomes where their cargo is degraded [133]. During microautophagy, the membranes of lysosomes invaginate and include cytosolic material directly. In contrast, chaperone-mediated autophagy describes the internalization of proteins into lysosomes via binding of targeted proteins to a chaperone.
Recently, it has been appreciated that autophagy plays a central role in the heart under physiological as well as under pathophysiological conditions. An excellent example is provided by the early postnatal period that is associated with starvation. Newborn mice exhibited a significant increase of the autophagic flux due to starvation [135]. Furthermore, autophagy was largely activated in mice that were subjected to experimental starvation for 1–3 days [136, 137]. Blocking autophagy in mouse models by deletion of genes encoding for central proteins of this degradation pathway such as beclin-1 [138], Atg5 [135], or Atg7 [139] resulted in embryonic or neonatal lethality. Interestingly, the pharmacological inhibition of autophagy by systemic application of bafilomycin A1, which prevents the fusion of autophagosomes with lysosomes, caused heart failure in mice [137]. Similarly, the inducible, heart-specific knockout of Atg5 in adult mice was associated with cardiac dilation and dysfunction. On the other hand, mice with an embryonic heart-specific deletion of Atg5 did not develop a heart failure phenotype, but were sensitized to pressure overload by aortic banding resulting in aggravated left ventricular dysfunction [140]. Induction of autophagy has been documented under hypertrophic conditions following aortic constriction [141]. However, reduction of the autophagic flux by haploinsufficiency of beclin-1 in heterozygous knockout mice improved cardiac function after aortic banding, while hypertrophy was similar to banded control animals [141]. The overexpression of beclin-1 in turn exacerbated hypertrophy upon pressure overload and was associated with diminished fractional shortening. Taken together, the published data so far do not resolve the function of autophagy in hypertrophy. Complete inhibition of autophagy is obviously detrimental. Excessive activation of autophagy seems to be a maladaptive mechanism as well though. Since it has been shown that autophagy plays a role in human cardiac disease as well, such as dilated cardiomyopathy [142], blocking autophagy in a controlled fashion might, thus, be an attractive target in the therapy of hypertrophy.
18.3.2 Noncoding RNAs in Cardiac Hypertrophy
18.3.2.1 MicroRNAs
In contrast to the well-known protein-coding messenger RNAs (mRNAs), there is an emerging world of noncoding RNAs (reviewed in [143]), providing a previously unrecognized layer of regulation of gene expression. Noncoding RNAs are divided into short (<200 bp) and long RNAs (>200 bp) due to technical reasons of RNA isolation. MicroRNAs are short RNAs (19–25 bp) that typically directly regulate posttranscriptional gene expression. Precursors of a mature microRNA, the so-called pri-miRNAs, are transcribed by RNA polymerase II. The pri-miRNAs form hairpin structures that are further processed by Drosha and DGCR8 into pre-miRNAs in the nucleus. After nuclear export into the cytoplasm, Dicer cleaves pre-miRNAs resulting in a double-stranded microRNA. One strand is incorporated into the RNA-induced silencing complex (RISC) as a mature microRNA [144]. Complimentary binding of the so-called seed region of the microRNA with the mRNA is sufficient to cause significant repression by degradation or translation inhibition of mRNAs. The binding sites are usually located at the 3′ untranslated region of mRNAs. Therefore, it is not surprising that a single microRNA can regulate many targets. Conversely, a single mRNA has several potential binding sites for different microRNAs. Presumably, different cardiac disorders are associated with the induction or repression of a distinct subset of microRNAs. During the past decade, attempts have been made to establish a signature of microRNAs that is associated with cardiac hypertrophy not only in mouse models [145, 146], but also in humans [147]. Of note, microRNAs are not present in cells only but can also be detected in plasma. These circulating microRNAs are surprisingly stable, because they are packaged in exosomes [148] and microvesicles [149] or carried by proteins such as Argonaute 2 (Ago2) [150] and high-density lipoproteins (HDL) [151]. Circulating microRNAs are currently evaluated as a novel group of biomarkers in clinical medicine. With respect to cardiovascular disorders, microRNAs have been analyzed as biomarkers in acute myocardial infarction, heart failure, and coronary artery disease [152] (Chap. 13).
Several mouse models have been generated that underline the importance of microRNAs in the pathogenesis of cardiac hypertrophy. One of the first microRNAs that has been implicated in hypertrophic growth was miR-208. miR-208a is located in intron 27 of the murine α-MHC (myosin heavy-chain, Myh6) gene. It is co-transcribed with α-MHC. The homozygous deletion of miR-208a resulted in viable mice that developed normally. Under stress conditions due to aortic constriction however, they lacked a hypertrophic response and the isoform switch from α- to β-MHC (Myh7) [153]. Conversely, cardiac overexpression of miR-208a causes hypertrophy and elevated β-MHC levels [154]. The main underlying mechanism is that miR-208 targets the thyroid hormone receptor-associated protein 1 (THRAP1). Thyroid hormone (T3) signaling induces the expression of α-MHC but represses the expression of β-MHC [155]. The T3-dependent regulation of MHC isoforms is disturbed in miR-208a knockout mice. A second and a third member of the miR-208 family, miR-208b [154] and miR-499 [156], have been identified within the β-MHC gene (Myh7/Myh7b) and have also been implicated in the pathogenesis of cardiac hypertrophy and heart failure [157, 158]. Subsequently, the therapeutic potential was tested with an anti-miR against miR-208a in a rat model of salt-sensitive hypertension [159]. Its systemic administration reduced cardiomyocyte hypertrophy and improved survival. The role of miR-499 is still controversial in the context of hypertrophy. A transgenic mouse model with overexpression of miR-499 exhibited cardiac hypertrophy and fibrosis [157]. Yet, another model of miR-499 did not develop spontaneous hypertrophy. The transgenic mice were even protected from the effects of ischemia and reperfusion [160]. Of note, in this study, calcineurin was identified as a direct molecular target of miR-499.
The muscle-specific microRNA miR-1 belongs to a cluster of microRNA genes consisting of miR-1-1/133a-2, miR-1-2/133a-1, and miR-206/133b [144]. miR-1 as well as miR-133 is downregulated in several models of hypertrophy. The genetic ablation of miR-1-2 results in embryonic lethality in 50 % probably due to ventricular septal defects (VSD) [161]. The surviving animals display ECG abnormalities and arrhythmias. Although the overexpression of miR-1 could mitigate hypertrophy in neonatal rat cardiomyocytes and blunt hypertrophy after injection of miR-1 into the heart of isoproterenol-stimulated animals [162], the overexpression of miR-1 in transgenic mice caused heart failure and hypertrophy [163]. The homozygous knockout of either identical microRNA miR-133a-1 or miR-133a-2 did not result in an obvious phenotype. The double knockout however caused increased embryonic lethality with animals displaying chamber dilation, wall thinning, and VSDs [164]. Only one-fourth of the double knockout mice survived and exhibited reduced cardiac function but no hypertrophy. However, adult mice that received an antagomir against miR-133 developed cardiac hypertrophy [165]. Interestingly, calcineurin also seems to be a target of miR-133 and in turn regulates the expression of miR-133 [166].
Besides the abovementioned microRNAs, also miR-199b modulates calcineurin/NFAT signaling [77]. The cardiac overexpression of miR-199b targets the kinase Dyrk1a, thereby causing exaggerated hypertrophy upon calcineurin overexpression in vivo or transverse aortic constriction. Overexpression of the miR-212/132 family causes cardiac hypertrophy and heart failure [167]. Conversely, a homozygous deletion blunted the effects of the pro-hypertrophic stimulus due to transverse aortic constriction. Again, the calcineurin/NFAT pathway seems to be the target of these microRNAs. miR-212/132 represses the transcription factor Foxo3 that modulates calcineurin activity [168] by regulating the expression of atrogin-1, an F-box protein. Atrogin-1 binds to Skp1, Cul1, and Roc1 assembling an SCF ubiquitin ligase complex that promotes ubiquitination of calcineurin and subsequent degradation [169]. Of note, the application of an anti-miR against miR-132 could prevent hypertrophy due to aortic constriction in vivo [167].
The outlined above examples show that microRNAs are promising targets of pharmacologic intervention by administering either microRNA mimics that amplify the effects of microRNAs or anti-miRs that inhibit gene regulation of microRNAs. For further information, we recommend excellent review articles about the role of microRNAs in the heart [170–172] and also see Chaps. 14, 15, and 62.
18.3.2.2 Long Noncoding RNAs
More recently, the focus on noncoding RNAs in cardiac biology has broadened from microRNAs to long noncoding RNAs. Long noncoding RNAs (lncRNA) are a heterogeneous group of RNAs with diverse functions. lncRNAs participate in transcriptional regulation by, e.g., chromatin modifications and posttranscriptional gene regulation by binding RNAs as well as by serving as scaffolds in macromolecular complexes [173]. So far, the lncRNA Fendrr has been implicated in heart development [174]. Moreover, another lncRNA termed braveheart seems to regulate cardiomyocyte differentiation [175]. Recently, lncRNAs were also connected to cardiac hypertrophy (Chap. 13). First attempts have been made to identify lncRNAs that are expressed or even enriched in the heart, but only a few were regulated during hypertrophy [176]. The specific lncRNA CHRF (AK048451) modulates hypertrophy by binding miR-489. The overexpression of miR-489 in a transgenic mouse model blunts hypertrophy and fibrosis due to chronic angiotensin II infusion. Myd88 (myeloid differentiation primary response gene 88) was identified as the target of miR-489. Consistently, Myd88 knockout mice were resistant to hypertrophy caused by angiotensin II as well. Mechanistically, CHRF seems to act as a sponge binding miR-489, thereby reducing miR-489 levels available for the regulation of gene expression [177].
Another cluster of lncRNAs is located within the Myh7 gene. These lncRNAs were named myosin heavy-chain-associated RNA transcripts (Myheart, or Mhrt) [178]. They are expressed in a heart-specific fashion and are enriched in the adult myocardium. In that study, Mhrts were repressed under hypertrophic conditions after aortic banding. The transgenic overexpression of a single lncRNA reduced cardiac hypertrophy and improved heart function in mice that were subjected to pressure overload. Mhrt inhibited hypertrophy by antagonizing BRG1, a member of chromatin-modifying enzymatic complexes. Of note, BRG1 itself regulates the transcription of Mhrt.
Although the field of lncRNA in cardiac biology and hypertrophy is very young, the above outlined examples highlight the importance of lncRNAs in the gene regulation network and emphasize the potential of lncRNAs as therapeutic targets.
18.3.2.3 Circular RNAs
Although circular RNAs were first described in eukaryotic cells in 1979 [179], their biological significance has been unknown. Originally, the formation of circular RNAs was thought to be an uncommon and rare event. However, RNA sequencing enabled the identification of numerous circRNAs [180]. Two recent studies discovered an important function of circRNAs in posttranscriptional gene regulation. It has been shown before that transcripts of the sex-determining region Y (Sry) gene form circRNAs. Interestingly, more than 90 % of the transcripts in adult mouse testes are circular [181]. Now, there is evidence that Sry serves as a “sponge” for miR-138 [182] due to multiple binding sites for miR-138. Moreover, the antisense transcript of cerebellar degeneration-related protein 1 (CDR1) termed CDR1as or ciRS-7 binds miR-7 like a sponge as well [182, 183]. Sponge RNAs inhibit the function of microRNAs merely by complementary binding. Accordingly, the expression level of target genes of miR-7 was affected by CDR1as/ciRS-7. Knockdown of CDR1as/ciRS-7 caused downregulation of target genes [182, 183]. Interestingly, the morpholino-mediated knockdown of miR-7 reduced the size of the midbrain in zebrafish. A similar phenotype was produced by injection of CDR1as/ciRS-7 [183]. The novel function of circRNAs adds another layer of complexity to posttranscriptional gene regulation and may provide further opportunities for therapeutic interventions also in the context of cardiac hypertrophy and heart failure.
18.4 Concluding Remarks
Cardiac hypertrophy predisposes toward increased mortality as an independent risk factor. Therefore, inhibition or regression of hypertrophy appears to be an attractive target for medical intervention. In line with this notion, multiple trials have shown that regression of left ventricular hypertrophy is associated with improved survival. The HOPE (“Heart Outcomes Prevention Evaluation”) study indicated that treatment with the ACE inhibitor ramipril reduced cardiovascular events such as myocardial infarction and stroke as well as mortality [184] associated with attenuation or regression of left ventricular hypertrophy as assessed by ECG [185]. Consistently, a subgroup analysis of the LIFE (“Losartan Intervention For Endpoint reduction in hypertension”) trial showed that reduction of the left ventricular mass measured by echocardiography decreases the risk for myocardial infarction, stroke, and mortality independent of blood pressure reduction [186].
Beyond the established inhibition of α- and β-adrenergic and angiotensin receptors, the discovery of additional intracellular pathways underlying pathological hypertrophy made it possible to consider specific molecular therapies. For example, several inhibitors of hypertrophy (as outlined above) might be delivered by a gene therapy approach in the future (Chap. 15). In order to achieve cardiac gene delivery, adeno-associated viruses (AAV) have been successfully employed in a number of animal models [187]. Recently, overexpression of SERCA2a via AAV1 in heart failure has been examined in a phase 2 trial [188]. However, due to remaining immunological issues that are associated with application of AAVs, the direct administration of RNA species as regulators of gene expression might be an interesting alternative. Dependent on the function of a specific microRNA, therapeutics can enhance or blunt the effect of microRNAs by using mimics or anti-miRs. Extensive work has been done on chemical modifications of microRNAs to enhance stability and binding affinity [172]. Furthermore, the therapeutic use of RNA sponges might be useful to negatively modulate microRNA effects.
Extensive molecular research has provided a variety of interventional opportunities. Upcoming therapies will have to be carefully evaluated not only with respect to efficacy but also with respect to patient safety. Additionally, new approaches will have to compete with the existing therapies. Nevertheless, there is a hope that patients will benefit from specific antihypertrophic therapies in the future.
References
1.
Li F, Wang X, Capasso JM, Gerdes AM. Rapid transition of cardiac myocytes from hyperplasia to hypertrophy during postnatal development. J Mol Cell Cardiol. 1996;28(8):1737–46. doi:10.1006/jmcc.1996.0163.PubMed
2.
Hill JA, Olson EN. Cardiac plasticity. N Engl J Med. 2008;358(13):1370–80. doi:10.1056/NEJMra072139.PubMed
3.
Frey N, Katus HA, Olson EN, Hill JA. Hypertrophy of the heart: a new therapeutic target? Circulation. 2004;109(13):1580–9. doi:10.1161/01.CIR.0000120390.68287.BB.PubMed
4.
de Simone G, Gottdiener JS, Chinali M, Maurer MS. Left ventricular mass predicts heart failure not related to previous myocardial infarction: the Cardiovascular Health Study. Eur Heart J. 2008;29(6):741–7. doi:10.1093/eurheartj/ehm605.PubMed
5.
Levy D, Anderson KM, Savage DD, Balkus SA, Kannel WB, Castelli WP. Risk of ventricular arrhythmias in left ventricular hypertrophy: the Framingham Heart Study. Am J Cardiol. 1987;60(7):560–5.PubMed
6.
Levy D, Garrison RJ, Savage DD, Kannel WB, Castelli WP. Prognostic implications of echocardiographically determined left ventricular mass in the Framingham Heart Study. N Engl J Med. 1990;322(22):1561–6. doi:10.1056/NEJM199005313222203.PubMed
7.
Fredriksson R, Lagerstrom MC, Lundin LG, Schioth HB. The G-protein-coupled receptors in the human genome form five main families. Phylogenetic analysis, paralogon groups, and fingerprints. Mol Pharmacol. 2003;63(6):1256–72. doi:10.1124/mol.63.6.1256.PubMed
8.
Lohse MJ, Engelhardt S, Eschenhagen T. What is the role of beta-adrenergic signaling in heart failure? Circ Res. 2003;93(10):896–906. doi:10.1161/01.RES.0000102042.83024.CA.PubMed
9.
Rockman HA, Koch WJ, Lefkowitz RJ. Seven-transmembrane-spanning receptors and heart function. Nature. 2002;415(6868):206–12. doi:10.1038/415206a.PubMed
10.
Rohrer DK, Desai KH, Jasper JR, Stevens ME, Regula Jr DP, Barsh GS, Bernstein D, Kobilka BK. Targeted disruption of the mouse beta1-adrenergic receptor gene: developmental and cardiovascular effects. Proc Natl Acad Sci U S A. 1996;93(14):7375–80.PubMedCentralPubMed
11.
Engelhardt S, Hein L, Wiesmann F, Lohse MJ. Progressive hypertrophy and heart failure in beta1-adrenergic receptor transgenic mice. Proc Natl Acad Sci U S A. 1999;96(12):7059–64.PubMedCentralPubMed
12.
Bisognano JD, Weinberger HD, Bohlmeyer TJ, Pende A, Raynolds MV, Sastravaha A, Roden R, Asano K, Blaxall BC, Wu SC, Communal C, Singh K, Colucci W, Bristow MR, Port DJ. Myocardial-directed overexpression of the human beta(1)-adrenergic receptor in transgenic mice. J Mol Cell Cardiol. 2000;32(5):817–30. doi:10.1006/jmcc.2000.1123.PubMed
13.
Milano CA, Allen LF, Rockman HA, Dolber PC, McMinn TR, Chien KR, Johnson TD, Bond RA, Lefkowitz RJ. Enhanced myocardial function in transgenic mice overexpressing the beta 2-adrenergic receptor. Science. 1994;264(5158):582–6.PubMed
14.
Du XJ, Autelitano DJ, Dilley RJ, Wang B, Dart AM, Woodcock EA. Beta(2)-adrenergic receptor overexpression exacerbates development of heart failure after aortic stenosis. Circulation. 2000;101(1):71–7.PubMed
15.
Du XJ, Gao XM, Jennings GL, Dart AM, Woodcock EA. Preserved ventricular contractility in infarcted mouse heart overexpressing beta(2)-adrenergic receptors. Am J Physiol Heart Circ Physiol. 2000;279(5):H2456–63.PubMed
16.
Liggett SB, Tepe NM, Lorenz JN, Canning AM, Jantz TD, Mitarai S, Yatani A, Dorn 2nd GW. Early and delayed consequences of beta(2)-adrenergic receptor overexpression in mouse hearts: critical role for expression level. Circulation. 2000;101(14):1707–14.PubMed
17.
Chruscinski AJ, Rohrer DK, Schauble E, Desai KH, Bernstein D, Kobilka BK. Targeted disruption of the beta2 adrenergic receptor gene. J Biol Chem. 1999;274(24):16694–700.PubMed
18.
Revelli JP, Preitner F, Samec S, Muniesa P, Kuehne F, Boss O, Vassalli JD, Dulloo A, Seydoux J, Giacobino JP, Huarte J, Ody C. Targeted gene disruption reveals a leptin-independent role for the mouse beta3-adrenoceptor in the regulation of body composition. J Clin Invest. 1997;100(5):1098–106. doi:10.1172/JCI119620.PubMedCentralPubMed
19.
Kohout TA, Takaoka H, McDonald PH, Perry SJ, Mao L, Lefkowitz RJ, Rockman HA. Augmentation of cardiac contractility mediated by the human beta(3)-adrenergic receptor overexpressed in the hearts of transgenic mice. Circulation. 2001;104(20):2485–91.PubMed
20.
Bristow MR. Beta-adrenergic receptor blockade in chronic heart failure. Circulation. 2000;101(5):558–69.PubMed
21.
Lymperopoulos A, Rengo G, Koch WJ. Adrenergic nervous system in heart failure: pathophysiology and therapy. Circ Res. 2013;113(6):739–53. doi:10.1161/CIRCRESAHA.113.300308.PubMed
22.
Dahlof B, Pennert K, Hansson L. Reversal of left ventricular hypertrophy in hypertensive patients. A metaanalysis of 109 treatment studies. Am J Hypertens. 1992;5(2):95–110.PubMed
23.
Bangalore S, Messerli FH, Kostis JB, Pepine CJ. Cardiovascular protection using beta-blockers: a critical review of the evidence. J Am Coll Cardiol. 2007;50(7):563–72. doi:10.1016/j.jacc.2007.04.060.PubMed
24.
Pitcher JA, Freedman NJ, Lefkowitz RJ. G protein-coupled receptor kinases. Annu Rev Biochem. 1998;67:653–92. doi:10.1146/annurev.biochem.67.1.653.PubMed
25.
Ungerer M, Bohm M, Elce JS, Erdmann E, Lohse MJ. Altered expression of beta-adrenergic receptor kinase and beta 1-adrenergic receptors in the failing human heart. Circulation. 1993;87(2):454–63.PubMed
26.
Jaber M, Koch WJ, Rockman H, Smith B, Bond RA, Sulik KK, Ross Jr J, Lefkowitz RJ, Caron MG, Giros B. Essential role of beta-adrenergic receptor kinase 1 in cardiac development and function. Proc Natl Acad Sci U S A. 1996;93(23):12974–9.PubMedCentralPubMed
27.
Matkovich SJ, Diwan A, Klanke JL, Hammer DJ, Marreez Y, Odley AM, Brunskill EW, Koch WJ, Schwartz RJ, Dorn 2nd GW. Cardiac-specific ablation of G-protein receptor kinase 2 redefines its roles in heart development and beta-adrenergic signaling. Circ Res. 2006;99(9):996–1003. doi:10.1161/01.RES.0000247932.71270.2c.PubMed
28.
Raake PW, Vinge LE, Gao E, Boucher M, Rengo G, Chen X, DeGeorge Jr BR, Matkovich S, Houser SR, Most P, Eckhart AD, Dorn 2nd GW, Koch WJ. G protein-coupled receptor kinase 2 ablation in cardiac myocytes before or after myocardial infarction prevents heart failure. Circ Res. 2008;103(4):413–22. doi:10.1161/CIRCRESAHA.107.168336.PubMedCentralPubMed
29.
Rockman HA, Chien KR, Choi DJ, Iaccarino G, Hunter JJ, Ross Jr J, Lefkowitz RJ, Koch WJ. Expression of a beta-adrenergic receptor kinase 1 inhibitor prevents the development of myocardial failure in gene-targeted mice. Proc Natl Acad Sci U S A. 1998;95(12):7000–5.PubMedCentralPubMed
30.
Freeman K, Lerman I, Kranias EG, Bohlmeyer T, Bristow MR, Lefkowitz RJ, Iaccarino G, Koch WJ, Leinwand LA. Alterations in cardiac adrenergic signaling and calcium cycling differentially affect the progression of cardiomyopathy. J Clin Invest. 2001;107(8):967–74. doi:10.1172/JCI12083.PubMedCentralPubMed
31.
Raake PW, Schlegel P, Ksienzyk J, Reinkober J, Barthelmes J, Schinkel S, Pleger S, Mier W, Haberkorn U, Koch WJ, Katus HA, Most P, Muller OJ. AAV6.betaARKct cardiac gene therapy ameliorates cardiac function and normalizes the catecholaminergic axis in a clinically relevant large animal heart failure model. Eur Heart J. 2013;34(19):1437–47. doi:10.1093/eurheartj/ehr447.PubMedCentralPubMed
< div class='tao-gold-member'>
Only gold members can continue reading. Log In or Register a > to continue
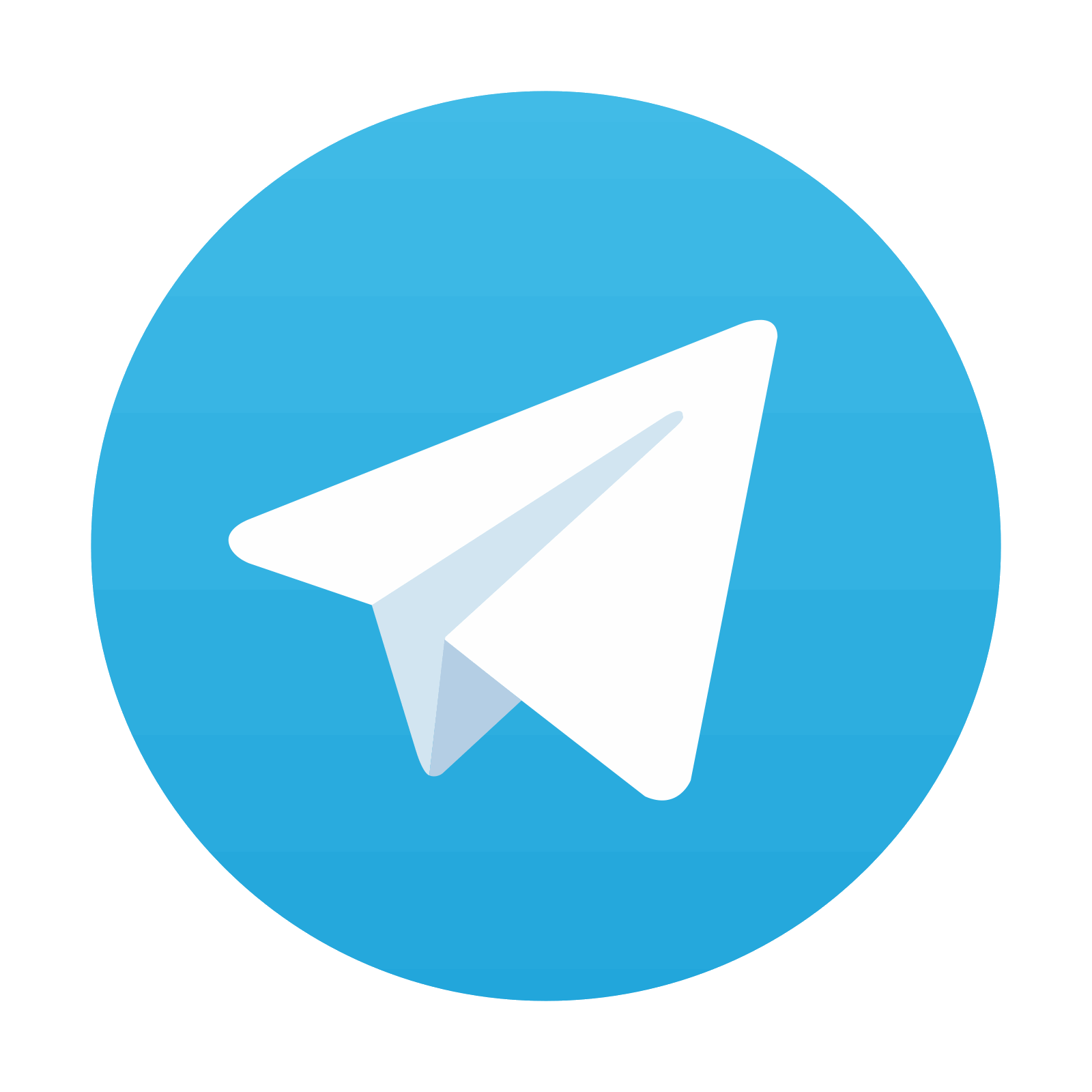
Stay updated, free articles. Join our Telegram channel
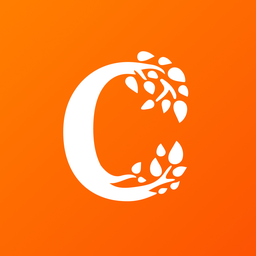
Full access? Get Clinical Tree
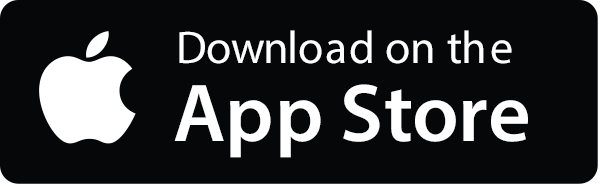
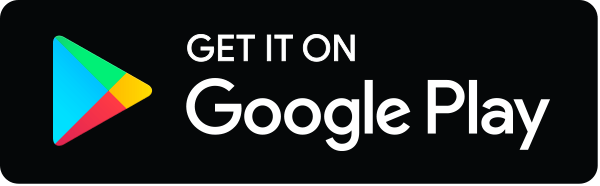