Fig. 57.1
Schematic representation of heart development in mice (upper row) and hypothetical depiction of factors leading to HLHS, as summarized from the literature (for references, see text). Abbreviations: OFT outflow tract, RV right ventricle, LV left ventricle, RA right atrium, LA left atrium, AO aorta, PA pulmonary artery, PS primitive streak, E embryonic stage in days postconception
Following the introductory part about left heart development, we will discuss the molecular pathways that are involved in HLHS. We will start from the early transcriptional programs that are established in the developing heart, proceed with the Notch signaling pathway, and discuss cell cycling and growth factor contribution to left heart hypoplasia. We will finish this part by considering epigenetic and immunological factors in HLHS. Subsequently, we will take a look at previous and current animal models used in researching HLHS and evaluate the difficulties in utilizing model systems for this rare syndrome. The chapter closes with a view ahead into the future challenges and opportunities for researchers and clinicians in the quest for improving our understanding of HLHS.
57.2 Heart Fields and Cell Lineages in HLHS
57.2.1 Origins of Ventricular Myocardial Cells: Cardiac Mesoderm and the First and Second Heart Field
Heart cells emerge at gastrulation stages and are clonally related mainly to endodermal and mesodermal lineages [7]. One of the earliest markers of cardiogenic cells is Mesp1, a basic helix-loop-helix (bHLH) transcription factor required for mesodermal cells to generate a linear heart tube after leaving the primitive streak [8]. However, Mesp1 expression is not restricted to cardiovascular lineages and acts mainly through inducing a combinatorial set of specific cardiogenic target genes in early precursor cells [9]. Other early regulators of cardiac specification of mesoderm include the T-box transcription factor Eomes [10], which is an activator of Mesp1, as well as the cell fate factors Numb and Numbl, which are regulators of an undifferentiated and expansive population of cardiogenic precursor cells [11].
Long viewed as the sole contributor of cells for the mature heart, the early linear heart tube does not contain the whole population of cells that later make up the adult heart. Different genetic labeling experiments now provide ample evidence that the cardiac crescent is made up of distinct cell populations called the first and second heart field [12, 13] (Fig. 57.1). Clonal analysis in mice revealed that cells from the first heart field contribute primarily to left ventricle, both atria, and the right ventricle but not to the outflow tract, whereas cells from the second heart field mainly contribute to the outflow tract, the right ventricle, and both atria, but not the left ventricle [13, 14] (Fig. 57.1). The second heart field itself can be divided into an anterior and posterior part. Elegant explant experiments in mice showed that cells from the anterior second heart field were attributed to the outflow tract and right ventricular myocardium, whereas cells from the posterior second heart field make up the atrial myocardium [15]. While the phenotype of Numb/Numbl double knockout mice seems to arise from a role of these two genes in the second heart field and therefore is not reminiscent of HLHS, it is intriguing to speculate that similar mechanisms exist for precursor populations destined for a left ventricular fate. Recent clonal analysis of the earliest cardiovascular progenitor cells revealed two temporarily distinct Mesp1 progenitor cell populations in the first and second heart field [9]. The identification of distinct origins of progenitor cells might suggest that primary growth defects of the left ventricle itself may arise from perturbations in the genetic program of a cellular subpopulation within the first heart field.
57.2.2 The Ballooning Model of Cardiac Chamber Formation
After its formation, the linear heart tube starts to loop and the previously ventrally located side of the heart, primarily fated to constitute the left ventricle, starts to enlarge, differentiate, and form the primitive left and right ventricle [16]. This local expansion of myocytes is known as the “ballooning model of chamber formation” [17]. Prior to the concept of “ballooning,” it was hypothesized that all segments of the linear heart tube were pre-patterned to become anatomic regions of the adult heart. In contrast, the current concept of heart development allows for contribution of several distinct cell lineages and localized expansion of these cell populations (for a comprehensive review of recent concepts in cardiac development, see [18]). Newly formed myocardial cells constituting the linear heart tube initially display slow proliferative properties [6, 19]. Cell expansion of chamber myocardium at the looping heart tube stage is achieved by a local increase in proliferation and cell size at the outer curvature leading to the formation of the ventricular chambers [20]. During this stage, the contractile machinery of the ventricles develops by cell differentiation at the outer curvature [21]. At the same time, the myocardium of the inner curvature, the outflow tract, and the atrioventricular canal remain in a less differentiated state. This local repression of differentiation is mediated by a set of T-box transcription factors specifically expressed by precursors of the non-chamber myocardium [22].
The cell cycle itself is by far less well understood in (cardiac) myocytes as opposed to most other cell types. Genes implicated in myocardial cell cycle regulation serve multiple processes essential for ventricular growth, such as cellular hypertrophy (e.g., cyclins and cyclin-dependant kinases (CDK) [23]), sarcomere integrity (e.g., myocardin (Myocd) [24]), and postnatal quiescence (e.g., pocket proteins Rb and p130 [25]). The same cell cycle mechanisms are required postnatal for hypertrophic growth and DNA synthesis [26]. In agreement with these observations, knockout models for several cell cycle regulators are embryonic lethal due to cardiac defects, either as constitutive or lineage-specific loss-of-function models [27].
57.2.3 The Endocardium, a Hemodynamic Sensor and Constitutive Component of the Developing Heart
The primitive heart tube consists of the inner, endocardial layer, and the outer myocardial layer, which come to lie in a sack formed by the thin pericardial layer. All three cardiac tissues arise from the same precursors in the epiblast [28]. Endocardium and myocardium are separated by cardiac jelly, produced by the myocardium. At looping of the linear heart tube, both the endocardium and myocardium simultaneously turn rightward on the ventral side of the heart. Hemodynamic factors like shear stress and retrograde flow actively modulate chamber formation at myocardial and endocardial levels in the ballooning outer curvature [29, 30]. The endocardium represents the flow-sensitive entity in the developing heart and regulates chamber morphogenesis through the modulation of cell size and growth via the hemodynamically sensitive transcription factor Klf2a [31]. Mechanical forces thus induce transcriptional programs and contribute to the regulation of myocardial chamber volume, endocardial cell morphology, and endocardial cell proliferation.
A blood flow-independent stimulant for cell proliferation at the growing outer curvature is bone morphogenetic protein (Bmp) signaling. In particular, Bmp10 is necessary for correct ventricular development. Bmp10 expression can be found in the developing murine heart as soon as E9.0 first at the trabeculated part of the ventricular chamber and later in the atrial wall [32]. In line with early expression patterns, Bmp10 knockout mice show a strong reduction of cardiomyocyte proliferative activity and elevated expression of p57Kip2, a cyclin-dependent kinase inhibitor that is implicated in inhibiting reentry of cardiomyocytes into cell cycling [33, 34]. Bmp signaling from the myocardium thus influences the proliferation of endocardial cells in collaboration with but unaffected by hemodynamic forces. In addition to hemodynamic and Bmp signaling, there is a tightly regulated growth balance between myocardial and endocardial cell lineages, as evidenced by the overgrowth of myocardium in the absence of Etv2, an ETS domain transcription factor required for endocardial development [35, 36]. The presence of the endocardial cell layer and its localized interaction with the myocardium present one of the key moments in the development of the ventricular chambers and therefore deserve increased attention in the search for the molecular origins of HLHS. In the following section, we will further discuss the molecular factors that participate in ventricular development and discuss their role in HLHS etiology.
57.3 Molecular Pathways in Left Heart Development
Development of the four-chambered heart is facilitated by a spatially and temporally tightly regulated network of transcription activators, repressors, and signaling cascades. Left heart structures are controlled by factors that act early during heart development as shown by the presence of left ventricular myocardial progenitors in the linear heart tube. The developmental differences between the right and left heart regions are reflected by chamber-specific factors that are exclusively expressed in cells of either the left or right side of the heart.
57.3.1 Ventricular Development Is Regulated by a Set of Specific Transcription Factors
Mesp1, a basic helix-loop-helix transcription factor (bHLH), is the earliest known cardiac marker and initiates the cardiac transcription factor cascade which will lead to the generation of cardiac mesoderm [8, 37, 38]. Mesoderm-derived progenitor cells of the first and second heart field become cardiac specific by expression of NK2 homeobox 5 (Nkx2-5), one of the earliest markers of cardiomyocyte differentiation [39–41]. Two factors that display exclusive right/left ventricular expression in the looping heart are Hand2 (dHand) and Hand1 (eHand), both bHLH transcription factors [42]. Although initial chick experiments using antisense oligonucleotide knockdown showed coexpression of both dHand and eHand in the linear heart tube, suggesting genetic redundancy, subsequent studies in mice revealed a differential expression pattern in the developing heart [43]. After the linear heart tube stage, dHand expression in mice is mainly found in the outflow tract and right ventricular cells, while eHand is found in the outflow tract and the early left ventricular structures [44]. The left ventricular contribution of eHand and the right ventricular contribution of dHand are also consistent with their spatial expression in the looping heart where eHand is solely found in the outer curvature [45]. Expression of eHand in turn is regulated by Nkx2-5 in mice, as the Nkx2-5 knockout mouse shows absence of eHand transcripts. Another important factor in ventricular development that is independent of eHand but directly regulated by dHand and Nkx2-5 is the Iroquois homeodomain transcription factor 4 (Irx4) [46]. Irx4 is expressed in mice from E7.5, where it is found in the cardiac primordia, to E11.5 when it is exclusively found in the myocardium of the ventricles. Irx4 contributes to ventricle chamber formation by activating ventricle-specific genes such as ventricular myosin heavy chain 1 (Myh15) and suppressing atrial-specific genes such as atrial myosin heavy chain 1 (Myh7) [47]. The early formation of the left ventricle is thus governed by the specific interaction of cardiac transcription factors that are spatially and temporally orchestrated and conserved throughout vertebrate evolution. Defects in this intricate system might contribute to the development of a hypoplastic left heart but more data will be necessary to evaluate the contribution of transcription factor defects to HLHS pathology in humans.
57.3.2 Notch Signaling at the Frontier of the Endocardium and Myocardium
The notch signaling pathway in cardiac development is best known for its role in outflow tract development, and multiple studies have associated mutations in the NOTCH1 receptor with aortic valve malformations [48–50]. The identification of NOTCH1 mutations in HLHS patients is therefore not very surprising, as HLHS is often thought to be secondary to outflow tract obstructions like BAV [51]. Notch signaling however might not only secondarily contribute to HLHS but also primarily by endocardial activation of myocardial trabeculation at the outer curvature of the ballooning heart [52]. Activation of Notch by endocardial-myocardial interaction has two distinct functions in the compaction of ventricular myocardium: (1) differentiation of compact and trabecular myocardium through EphB4/EphrinB2 endocardial-mediated Neuregulin 1 (Nrg1) activation, which in turn causes EphB4/EphrinB2 signaling activation in the myocardial cell layer [52]; and (2) inducing Bmp10 signaling at the growing poles of trabecular myocardium to provide a proliferative pool of cardiomyocytes. The control of these two processes by activated Notch is negatively regulated by Fkbp1a (Fkbp12), a peptidyl-prolyl cis-trans isomerase that is expressed ubiquitously and required for cardiac morphogenesis/homeostasis [53–55]. The effects that disrupted Notch signaling can impose on the developing ventricular system are manifold, and more research will be necessary to completely understand its developmental contribution to HLHS.
57.3.3 Cell Cycle Regulation and Ventricular Growth
As highlighted earlier in this chapter, myocardial progenitor cells are initially small, highly proliferative cells that in mammals lose the ability to divide and are arrested in their cell cycle progression at postnatal stages. Defects in cell cycle regulation have been associated with HLHS, and several studies have investigated differential gene expression in hypoplastic left hearts. Interestingly, gene expression analysis using microarray and qPCR experiments on human atrial septum samples from HLHS patients and cardiac surgery patients not affected by HLHS revealed differential expression of numerous cell cycle regulators and chromatin remodeling factors [56]. Proper cardiomyocyte differentiation and proliferation seems to be impaired by the upregulation of cell cycle repressors WEE1 G2 checkpoint kinase (WEE1), RNA binding motif, single-stranded interacting protein 1 (RBMS1), and numerous CDK inhibitors, which interfere with the transition between cell cycle states [56, 57]. Another layer of the transcriptional program in HLHS is mediated by factors regulating chromatin remodeling and chromatin structure. Increased expression of the histone deacetylase 2 (HDAC2) and MYND domain-containing transcription factor (SMYD1) that can cooperatively regulate activation and repression of cardiac specific factors like HAND1 and IRX4 indicate transcriptional repression in the HLHS atrial septum [58]. These studies were limited however by the fact that gene expression was only measured in atrial septum of HLHS infants but not in other cardiac structures. Differences found in the atrial septum might therefore not reflect expression patterns that contribute to left ventricular development in HLHS. Nevertheless, experimental evidence points to an important role of myocyte cell cycling at an early stage of myocyte differentiation in the pathogenesis of HLHS [5]. The molecular origins of these proliferation defects in the developing human heart however remain enigmatic for now.
57.3.4 Epigenetic and Immunologic Contributions to HLHS
In addition to the genetic contributors enumerated above, other factors may play synergistic roles in the etiology of HLHS. Maternal immune response has recently been proposed to be a possible cause of HLHS [59]. According to this model, antibodies produced by the pregnant mother in response to infection are transmitted through the placenta to the fetus, where they damage the developing heart, either directly or indirectly by affecting the blood flow in the forming chambers. This hypothesis was recently tested using a fetal rat immunization model [60]. Offspring of females that were immunized with cardiac myosin (CM) prior to pregnancy showed a high burden of left-sided structural congenital malformations, of which hypoplasia of the left ventricular cavity was the most common one. The malformed development of left heart structures in this model could be interpreted in two different ways: (1) possibly, autoimmune-mediated mechanisms could impede left ventricular growth through direct cellular damage. Given the scarcity of recurrence of HLHS, however, this seems less likely. (2) Inhibition of sarcomere function during left ventricular development, such as through impaired systolic or diastolic properties, may lead to secondary cellular growth defects. This mechanism could also hold true in the absence of direct medical relevance of an autoimmune-mediated process in human HLHS.
One of the major limitations in studying extremely rare cardiac malformations like HLHS has always been the lack of specific cell culture models due to the difficulty in obtaining primary tissue. Recent advances in stem cell biology have empowered researchers and clinicians with the ability to reprogram differentiated cells into induced pluripotent stem cells (iPSCs) [61]. Two recent studies utilizing this technology found that iPSCs derived from HLHS patients show decreased cardiomyocyte differentiation potential compared to controls accompanied by repression of the major cardiac transcription factor genes NKX2-5, T-box (TBX) 2, the Notch/Hey signaling pathway, and significantly lower levels of heart and neural crest derivative-expressed (HAND) transcripts [62, 63]. Inhibition of NKX2-5 transcriptional activation was mediated by reduced methylation of histone marks H3K4 and H3 acetylation but an increased methylation of H3K27 marks on the NKX2-5 promoter region. These two independent findings suggest that repression and activation of key cardiac regulators orchestrates ventricular chamber formation in a structural and temporal manner and thus contributes to the molecular identity of HLHS.
57.4 Animal Models of HLHS
Animal models have been used extensively to study congenital heart malformations and gain insights into the general development of the heart. While a variety of different species have been utilized to study cardiovascular malformations, there are mainly three widely used established models, the zebrafish, the mouse, and the chick. Each of these models has certain characteristics advantageous for different lines of investigation. Unknowns in the etiology of HLHS present major challenges for researchers trying to establish model systems to investigate the onset and progression of disease. Conceptually, it is evident that the drawback of the zebrafish model to study hypoplasia of the left heart is its two-chamber architecture, with the lack of a specific left ventricle. However, ease of experimental manipulation, the capacity to examine myocardial regeneration, and the tight molecular conservation of vertebrate cardiogenesis make the zebrafish an indispensable model to study underlying processes of ventricular growth and regeneration [64–66]. Mice and chicken on the other hand show orthologous architecture in comparison to the human heart, making them excellent candidates for the investigation of HLHS.
57.4.1 The Chicken Heart as a Model for Hypoplasia of the Left Heart Structures
Chickens are evolutionarily further from humans than mammals like mice and rats, but they essentially share identical molecular and morphological cascades of heart development [67]. The similarities in chamber formation therefore enable the investigation of molecular patterns of human congenital heart malformations like HLHS in the chick. The first and currently only viable model system to investigate the progression of HLHS was created almost four decades ago using left atrial ligation [68]. Utilizing a nylon device in the region of the left atrioventricular canal and restricting blood flow to the developing left ventricular chamber, it was possible to recreate morphogenetic changes commonly seen in human HLHS patients. Especially interesting is the close resemblance of malformations like mitral valve atresia and premature closure of the foramen ovale to the human conditions seen in HLHS. These findings reinforced the idea of “no flow-no grow” in the developing heart chambers and provided a first basis for further investigation of hypoplastic left heart development. A more recent study utilized this model of restricted blood flow to show the impact of ventricular blood load on myocyte proliferation [69]. In addition to ligating the left atrioventricular canal, the authors also partially clipped the right atrial appendage to increase the blood flow to the left ventricle. Following this adjustment in blood flow, control as well as HLHS clipping model hearts showed an increase in myocyte proliferation in left heart structures. Developing chamber myocardium thus exhibits a certain plasticity that can be triggered by changes in ventricular load during development even in already hypoplastic circumstances. Hypoplasia of the ventricles early in development thus seems to be a reversible process highlighting the possibility for intervention at an early stage in the human embryo. As a clinical vignette, this approach has been successfully exploited in selected cases of severe left ventricular outflow tract obstruction in human fetuses, which have benefited from catheter-based treatment to restore normal flow through the left ventricle, with secondary improvement of ventricular growth [70]. Hemodynamically induced hypoplasia in chicken hearts, however, only mimics the mechanical forces and does not reflect intrinsic molecular defects triggering the development of HLHS in humans. It does however provide a basis for studying cellular responses of hypoplastic structures and the development of new treatment and diagnostic strategies.
57.4.2 The Absence of Murine Models Highlights the Complexity of HLHS
The complexity and oligogenic origin of HLHS becomes most evident by the fact that despite the extensive large-scale efforts in describing knockout mouse models [71], there is as yet no single reproducible mouse model for isolated HLHS. Neither forward nor reverse genetic approaches have proven successful to date. Large-scale ENU mutagenesis screens in mouse did not recover monogenic HLHS models, although one polygenic model has been reported [72] (Mouse Mutant Resource Web Site, The Jackson Laboratory, Bar Harbor, Maine. www.informatics.jax.org/reference/J:175213, [Feb 2015]). Knockout studies of Hand1, Hand2, and many others in mice offered important insights about the importance of these factors in ventricular development, but do not replicate HLHS per se [73]. Rescue of these embryos by contribution of wild-type trophoblasts leads to subsequent cardiac failure at E10.5 due to absent looping and cardiomyocyte differentiation defects. Absence of Hand1 during heart development leads to absence or severe hypoplasia of the left ventricle [73]. Unfortunately, the Hand1 mouse model as well as other models are embryonic or early postnatal lethal and do not replicate the progressive nature of HLHS. Several viable knockout mouse models however have been described to exhibit heart malformations that replicate certain features of HLHS, including several models with aortic stenosis (ADAM metallopeptidase domain 19 (Adam19), Notch1, GATA binding protein (Gata) 5, nitric oxide synthase 3 (Nos3), Nkx2-5, epidermal growth factor receptor (Egfr)) [48, 74–79].
57.4.3 Mechanical Flow Alterations in Fetal Lambs as a Proxy for Human HLHS
Studies performed in non-model organisms often utilized mechanical alteration of blood flow in an early developmental stage as a means to introduce development of HLHS like ventricular structures. In one of the earliest of such studies, ventricular blood flow in fetal lambs during midgestation was altered through partial obstruction of left ventricular inflow or outflow [80]. Lambs with LV inflow obstruction showed decreased weight ratio of LV/RV as well as a decrease of about 50 % in ventricular mean volume, mimicking the left ventricular HLHS phenotype in humans. Obstruction of the LV outflow tract showed a similar trend with a decrease in left ventricular volume and an increase in wall thickness. Interestingly, an artificial increase in LV afterload in this model caused hyperplasia of the left ventricular myocytes and thereby a large gain of LV mass. These findings hint at a more complex concept than “no flow-no grow” and highlight the ability of ventricular myocytes to induce localized proliferation due to external signals like shear stress and tension. While some interesting observations were made by simulating HLHS development using the fetal lamb model, there are major disadvantages to it. Experimental manipulation, generation time, and cost of the studies are just a few of the problems that are associated with large animal models. Furthermore, the induction of the hypoplastic left heart in this model is solely due to an alteration of blood flow by a single morphological cause, disregarding other factors such as genetic and environmental factors. The fetal lamb model of HLHS therefore remains limited in its power as a translational model of human HLHS.
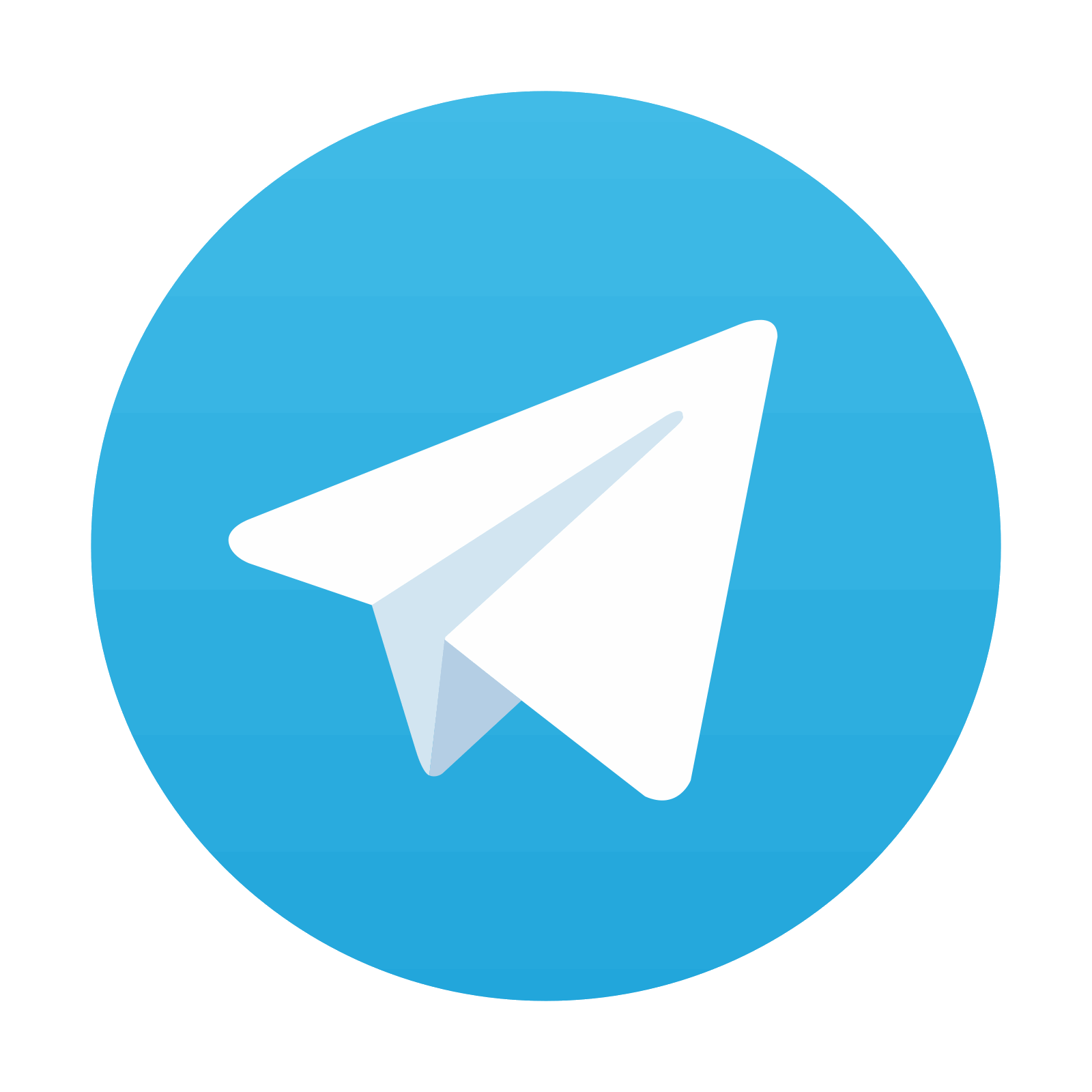
Stay updated, free articles. Join our Telegram channel
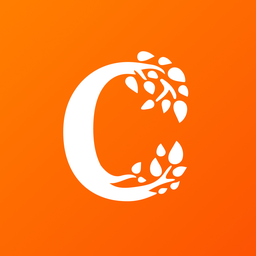
Full access? Get Clinical Tree
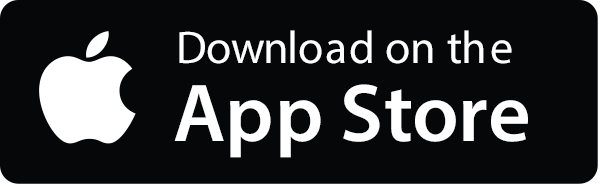
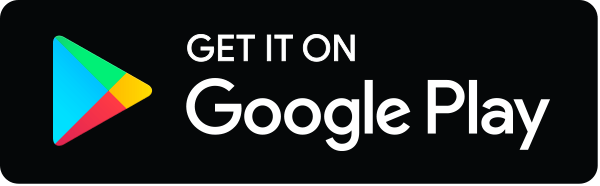