© Springer International Publishing Switzerland 2017
Andrea Ungar and Niccolò Marchionni (eds.)Cardiac Management in the Frail Elderly Patient and the Oldest Old10.1007/978-3-319-43468-1_33. Molecular Mechanisms of Cardiovascular Aging
(1)
Division of Geriatric Cardiology and Medicine, Research Unit of Medicine of Ageing, Department of Clinical and Experimental Medicine, University of Florence, Florence, Italy
(2)
National Institute on Aging, Baltimore, MD, USA
A number of functional and anatomical changes occur in the heart and vessels with age.
In human arteries, aging is characterized by structural modifications of endothelial and smooth muscle cells, forming the vascular wall. The hallmark of age-dependent endothelial dysfunction is an impaired endothelium-dependent vasodilatation. Aging is associated with reduced bioavailability of NO, due to decreased expression and activity of eNOS and deficiency of NOS substrates and cofactors [1]. Senescence is characterized also by intimal proliferation, increased arterial stiffness, and appearance of low grade, chronic inflammation [2–4].
Aging vessels show a defective capacity to repair that may be attributed to a loss in function of circulating endothelial progenitor cells (EPCs) [5]. This translates in defective re-endothelization of injured arteries [6].
In the heart, aging is identified by left ventricular hypertrophy, cardiac fibrosis, impaired ventricular compliance, progressive myocardial remodeling and deteriorated cardiac reserve. There is an increased sensitivity to stress, linked to decreased defensive mechanisms (e.g., ischemic preconditioning), resulting in heightened vulnerability to injury. The number of cardiomyocytes declines, in parallel with a progressive decline in organ regenerative capacity [7].
Intrinsic aging may be defined as the progressive structural and functional changes developed with age. However, it is a real challenge to discern the effects of aging per se from the modifications produced by the presence of cardiovascular risk factors, as “healthy” aging and “pathological” aging are deeply interrelated.
What is the molecular base of cardiac and vascular aging? The answer is complex. A multitude of pathways and signals are modified over time, all contributing to the aging phenotype. However, some senescence-related molecular mechanisms have been explored extensively and seem to give a distinct contribution to the age-related modifications detected in the cardiovascular system. Those pathways will be reviewed in this chapter.
3.1 Mitochondria and Cardiovascular Aging
Mitochondrial function is essential for the cell; mitochondria are the major source of energy, provide biosynthetic pathways, regulate differentiation and apoptosis, and control ion and redox homeostasis. Mitochondria provide energy for the cell in the form of adenosine triphosphate (ATP), by oxidative phosphorylation. Reactive oxygen species (ROS) are a natural byproduct of oxygen metabolism and the mitochondrial respiratory chain is the major source of ROS within the cell. An imbalance between production of oxidative molecules and ability of the cell to mount an appropriate antioxidant defense may result in oxidative damage to the main cellular structures.
The free radical theory of aging proposed by Harman in 1956, postulates that intracellular ROS are the major determinant of lifespan in mammals: age-related decline in organ functions could be ascribed to the detrimental effect of ROS on all major cellular components [8]. Since 90 % of cellular ROS are produced in mitochondria, Harman later hypothesized that mitochondrial ROS is mainly accountable for human aging [9]. In addition, due to the proximity of mitochondrial DNA (mtDNA) to ROS and the lack of protective histones, mtDNA is largely exposed to oxidative damage [10]. A “vicious cycle” hypothesis has been postulated which suggests that the initial ROS-induced damage to the mitochondrion increases the production of ROS that, in turn, leads to further mitochondrial damage.
However, it is well established that ROS are not purely harmful; they act as signaling molecules to modulate many intracellular pathways and their presence within the cell is crucial to build a strong response against stress (mitohormesis). Thus, while high levels of ROS are detrimental, the production of low levels of ROS may activate compensatory signaling pathways that promote longevity [11].
Several experimental evidence indicate that mitochondrial integrity declines as a function of age, resulting in an amplified leakage of ROS [12]. However, whether mitochondrial oxidative phosphorylation is impaired in senescence cells remains controversial. Some studies demonstrated a decline in oxygen consumption whereas others reported no change. This discrepancy may be due to the fact that there are two types of mitochondria: interfibrillar mitochondria exhibit a lower oxidative phosphorylation rate with age, whereas subsarcolemmal mitochondria, obtained from aged hearts, do not [13].
In the body as a whole, aging is accompanied by a decline in skeletal muscle mass and contractile function, leading to impaired mobility. However, this age-related decline in physical activity does not fully explain the decreased aerobic metabolic capacity detected in elderly individuals, as exemplified by trained older adults and master athletes still showing a reduced muscle and whole body VO2max. This can be attributed to an age-related, intrinsic decline in mitochondrial enzyme activity and function [14].
Among the five electron transport chain complexes, complex I includes seven of 13 mtDNA-encoded polypeptides. Therefore, complex I is likely to be the most susceptible to age-associated decline in activity and the major source of the increased ROS production with age [15]. Indeed, a recent study of aged mouse heart demonstrated an increased oxidative protein modification in complexes I and V, associated with a decline in energy-producing activity [16].
What is the evidence supporting the notion that age-related mitochondrial dysfunction contributes to cardiovascular aging?
Since the bulk of ROS production results from mitochondrial oxidative phosphorylation and myocardial activity is highly energy-dependent, the heart is particularly exposed to oxidative injury [17, 18].
The most convincing evidence that mitochondria and oxidative stress are related to cardiovascular aging originates by the study of transgenic animals. Mice overexpressing the antioxidant enzyme human catalase targeted to the mitochondrion (MCAT), exhibit a decreased level of oxidative damage and mtDNA deletions, and an increased lifespan. They also display a sharp protection from cardiac aging: compared to wild type mice, aged MCAT mice show reduced left ventricular mass index (LVMI), improved diastolic function (Ea/Aa), and reduced left atrium dilatation [19].
Mice expressing a proofreading-deficient version of mtDNA polymerase γ (PolG) accumulate a high number of mtDNA mutations and deletions in the heart and die prematurely from dilated cardiomyopathy [20]. They display early-onset morphological and functional changes to the heart, including fibrosis, cardiac enlargement, and impaired systolic and diastolic function. Cardiomyocytes of PolG mice exhibit accumulation of enlarged, irregularly shaped mitochondria, with reduced ATP production [21]. Of interest, PolG mice subjected to endurance exercise show improved mitochondrial biogenesis and mitochondrial morphology, and diminished level of apoptosis in multiple tissues and organs. Exercise also prevents premature mortality in this animal model [22]. Exercise may prove effective in mitigating age-related mitochondrial dysfunction in humans, as well.
Additional evidence of mitochondrial involvement in cardiac aging comes from mice with p66Shc gene mutation [23]. The p66Shc gene encodes a mitochondrial enzyme involved in the regulation of redox homeostasis; the mutation reduces ROS-mediated oxidative damage, modifies ROS-mediated intracellular signaling and prolongs the lifespan of mice. The mutation also increases cardiomyocyte resistance to apoptosis and prevents angiotensin II-induced LV hypertrophy [24, 25].
In the vasculature, the senescent phenotype is characterized by increased oxidative stress [26] and pro-inflammatory gene expression [2]. Generation of ROS within the vascular wall has been proposed to be the major pathophysiological mechanism of vascular aging. The production of mitochondrial ROS increases in senescence endothelial cells and VSMCs, in vitro and in vivo [27]. It has been suggested that increased production of ROS may contribute to generate the low-grade inflammation detected in aged vascular cells. Indeed, the increased NFkB activity observed during aging can be attenuated by H2O2 scavengers [28]. In addition, recent evidence suggest that endothelial cells may acquire a “senescence-associated secretory phenotype” which coordinates the synthesis and release of several proteins involved in the pro-inflammatory and pro-thrombotic pathways, including Interleukin-1α, ICAM-1, PAI-1 [29].
Mitochondrial Turnover and Aging
During the life of a cell, damaged mitochondria are continuously removed and replaced with newly formed organelles. This quality-control mechanism is necessary to maintain a functional mitochondrial pool. Only recently we discovered that mitochondria are fluid entities working in networks. For instance, the function of damaged mitochondria can be restored by their fusion with neighboring intact organelles [30]. If severely damaged, mitochondria are removed from the network by fission (break down into pieces of manageable size for engulfment) and eliminated by autophagy (mitophagy). Autophagy is a cytoprotective mechanism involving degradation and recycling of damaged intracellular organelles and proteins [31]. This mechanism is particularly important for the permanently differentiated cells, such as cardiomyocytes, which rely on autophagy to remove damaged cellular components. As cardiomyocytes are heavily dependent on mitochondrial oxidative metabolism for energy supply, the maintenance of a healthy pool of mitochondria is vital for the viability of these cells.
Recently, autophagy has emerged as a crucial regulator of the aging process [32]. The efficiency of autophagy seems to decrease with age [33, 34]. When mitochondrial turnover is unbalanced, the result is an accumulation of dysfunctional mitochondria which produce high levels of ROS and compromise the respiratory capacity of the cell [35].
The best indication that impaired autophagy/mitophagy is related to cardiovascular aging comes from transgenic animal studies. Autophagy impaired, Atg (autophagy-related) 5-deficient mice exhibit left ventricular dilatation, contractile dysfunction and heart failure, compared to control mice [36]. Mice with deficient autophagy/lysosome degradation mechanisms, activate an inflammatory response in cardiomyocytes when exposed to pressure overload [37]. Because cell-autonomous inflammation is a hallmark of cardiovascular aging [38], these data suggest that age-related alteration of mitochondrial autophagy may be causally linked to age-related cardiac inflammation.
Impaired mitochondrial biogenesis and reduced mitochondrial mass have been observed in senescent endothelial cells and VMSCs [39, 40]. A recent study involving older adults demonstrated that autophagy is impaired in aging human arteries and contributes to the endothelial dysfunction; enhancing autophagy reduces oxidative stress and inflammation and increases NO bioavailability, preserving a healthy endothelium [41].
Enough scientific evidence has been collected to indicate that optimization of autophagy in the aging heart may be beneficial against senescence and age-related cardiovascular diseases. Research is currently ongoing to screen for molecules able to enhance autophagy.
3.2 Telomeres and Cardiovascular Aging
Loss of replicative competence is the hallmark of cellular senescence and has been linked to telomere shortening [42]. Telomeres, repetitive DNA sequences located at the end of chromosomes, act as protective caps, preventing erosion of genetic material and fusion with neighboring chromosomes. Each time a cell divides, telomeres shorten. When telomeres become too short, the chromosomes can no longer be replicated and the cell enters senescence. Telomerase is a reverse transcriptase enzyme able to maintain the length of telomeres by adding DNA sequence repeats at the end of chromosomes. However, telomerase is poorly express in the majority of somatic cells [43].
Is telomere shortening implicated in cardiovascular aging?
Longitudinal cohort studies have related shorter telomeres in peripheral blood leukocytes to increased incidence of atherosclerotic disease [44]. If we compare morphologically normal arteries with diseased arteries, we find a significant difference in telomere length, with diseased vessels displaying shorter telomeres [45]. Intimal cells of iliac arteries have greater rate of telomere loss than internal mammary arteries, a difference that increases with age [46]. This variance in telomere length has been attributed to a greater hemodynamic stress, inducing higher telomere attrition, in iliac compared to mammary arteries. Telomere attrition and subsequent cellular senescence have been related to the propensity of iliac arteries to develop atherosclerotic lesions [46]. However, it is difficult to prove that telomere shortening is the reason for arterial disease; indeed, atherosclerosis per se may increase the proliferative index of vascular cells, signaled by reduced telomere length. Nevertheless, the senescent endothelial phenotype of human coronary arteries may be reversed by telomerase activity [47]. Of interest, in humans, physical exercise correlates with the maintenance of telomerase activity and telomere length, in circulating leukocytes and in vascular cells [48].
The human heart has been traditionally considered a post-mitotic organ. However, we know now that stem/progenitor cells with the ability to generate new cardiomyocytes exist in adult cardiac tissues [49], together with a subpopulation of myocytes that are not terminally differentiated and are still able to enter the cell cycle and undergo division [50]. The presence of cell division in non-diseased myocardium implies a turnover, although limited, of cells during the life span of the organism. An age-associated decline in stem/progenitor cell-dependent regeneration, associated with telomere shortening, may contribute to cardiac aging. Indeed, although mitotic index and telomerase activity increase with age, they are inadequate to compensate for cardiomyocyte loss in the adult heart [51]. Studies on humans have demonstrated that terminally differentiated cardiomyocytes are characterized by dysfunctional telomeres, as well. In post-mitotic cells, telomere-mediated signaling works in ways different than proliferative arrest. Indeed, telomere dysfunction may result in metabolic changes involving impaired mitochondrial biogenesis and electron transport chain performance, negatively affecting cardiac ATP synthesis and availability [52]. Therefore, age-associated telomerase dysfunction may instigate mitochondrial decay, which, in turn, may lead to decreased mitochondrial efficiency and increased ROS production.
In telomerase-deficient transgenic mice, telomere shortening is coupled with decreased cardiomyocyte proliferation, increased apoptosis, and cardiac remodeling resembling the phenotype of human aging heart [53]. Cardiomyocyte-specific reactivation of telomerase induces cardiac hypertrophy but not fibrosis or impaired function [54].
Taken together, these data suggest that telomere shortening may play a critical role in cardiac aging and may represent a promising target for therapy. In this regard, the demonstration that telomere dysfunctional mice can be rejuvenated by reactivation of endogenous telomerase [55], opens a scenario of possible future interventions on the aging heart.
3.3 Caloric Restriction, Sirtuins and Cardiovascular Aging
Caloric restriction (CR) is defined as a dietary regimen of limited energy intake in the absence of essential nutrients and vitamins deficiency. CR increases the median and maximal lifespan of all animal species tested, from yeast to mammals [56], including non-human primates (rhesus monkeys) [57], linking metabolism to aging.
Whether CR extends life span in humans will be never determined in randomized clinical trials for the obvious ethical implications and the limitation to follow study subjects until the end of their life. However, lower-length trials (from few months to few years) have been designed to determine the effect of CR on surrogate biomarkers of longevity. The randomized controlled trial CALERIE (Comprehensive Assessment of Long term Effects of Reducing Intake of Energy) was launched to test the health benefits of 2 years of intensive calorie restriction in human subjects [58]. However, the interpretation of the study results is controversial and there is still no conclusive indication whether or not reduced energy intake positively modulates longevity in humans.
Although there is no direct proof that CR is able to increase the mean and maximum lifespan of humans, it is widely accepted that reducing energy intake delays the aging of the cardiovascular system, through its pleiotropic effect on heart and vessels [59]. CR protects arteries from oxidative stress and fibrosis [60], prevents intima-medial thickening and inhibits the formation of atherosclerotic plaques [58]. Moreover, CR reverses vascular endothelial dysfunction by increasing the bioavailability of NO [61].
The positive effect of CR on the cardiovascular system is due, at least in part, to its effect on major cardiovascular risk factors: in human subjects exposed to reduced calorie intake total serum cholesterol, LDL cholesterol, total cholesterol/HDL cholesterol ratio, and triglycerides fall significantly; in addition, blood pressure, fasting glucose and C-reactive protein concentrations are extremely low, compared with age and sex-matched controls [58, 62]. Interestingly, CR has been shown to reduce myocardial collagen and extracellular matrix content [63] and to prevent LV diastolic dysfunction of the aging human heart [64].
Several hypotheses have been proposed to explain the positive effect of CR on cellular senescence, on the molecular level. The most intuitive hypothesis is that it regulates the exposure of the cell to oxidative stress [65]. It is well established that CR delays the age-related deterioration of mitochondrial function, limiting the surge of ROS production by the aged mitochondrion, and preserving the enzymatic activity of the electron transport system [66].
However, the link between limited energy intake and longevity is exquisitely complex [67]. Indeed, the longevity response to CR seems to be regulated by several nutrient-sensing signaling pathways, including mammalian target of rapamycin (mTOR) [68], insulin/IGF-1, and sirtuins (SIRT). All these pathways mediate a variety of cellular metabolic responses and control mitochondrial function. Of those, the most widely studied is mTOR.
TOR is a serine-threonine protein kinase that is inhibited by rapamycin and constitutes an important nutrient sensing pathway for the cell. It has been reported that inhibition of TOR activity induces longevity in yeast [69], C. elegans [70], and D. melanogaster [71]. Inhibition of mTOR with rapamycin dramatically extends the lifespan of mice [72], as well. mTOR integrates signaling from several intracellular nutrients and nutrient-sensitive pathways, including amino acids, insulin, growth factors, energy status, stress, and hypoxia. Downregulation of mTOR induces autophagy and mitophagy, improving the quality of mitochondrial function [73]. Chronic cardiac-specific activation of Akt stimulates mTOR and inhibits autophagic activity of myocardial cells, mimicking age-specific functional changes of the heart, including hypertrophy, fibrosis, decrease contractility and compromised diastolic function [74]. As Akt activity is enhanced by age, the Akt/mTOR pathway may play an important role in human heart aging.
Many studies report that CR reduces circulating levels of IGF-1 [75]. IGF-1 mediates several growth hormone (GH) biological responses and affects multiple signaling cascades, promoting proliferation, inhibition of apoptosis and stimulation of growth in many tissues and organs. The decreased level and activity of IGF-1/insulin observed under CR, have been causally linked to the lifespan increase of calorie restricted animals [76]. However, the effect of IGF-1/insulin signaling pathway on longevity is complex and somehow controversial [67]. Ames dwarf mutant mice which lack GH and have reduced IGF-1, live longer than control mice. These animals display potentiated anti-oxidant defenses. On the contrary, animals that overexpress GH have shorter lifespan than wild type animals, and display defected anti-oxidant capacity [77]. Mitochondria from Ames dwarf mice produce less ROS whereas GH transgenic mice exhibit greater protein oxidative damage, compared to wild types [78]. These data suggest that the effect of IGF-1 signaling on longevity may be mediated, at least in part, by its effect on oxidative stress. An alternative mechanism by which IGF-1 signaling may affect lifespan is the stimulation of autophagy [79]. However, it is important to emphasize that whereas CR reduces circulating levels of IGF-1 in most animal models, this is not true in men [80].
The effect of IGF-1 on life expectancy in humans is exquisitely complex. Low IGF-1 predicts extended life expectancy in exceptionally long-lived females [81]. However, IGF-1 deficiency is associated with premature atherosclerosis and elevated cardiovascular disease mortality [82]. IGF-1 protects cardiomyocytes from apoptosis, promotes cardiac stem cell survival, and improves myocardial contractile function [83, 84]. In the vasculature, IGF-1 counteracts the age-related carotid artery intima-media thickness [85]. On the other hand, higher IFG-1 levels are positively correlated with cancer mortality [86].
To further complicate things, recent data suggest that IGF-1 axis bioactivity, rather than IGF-1 serum concentration per se, is more likely to predict the effect of this hormone on cardiovascular health and mortality [87]. Therefore, the role of IGF-1 on life expectancy in humans remains elusive.
The data supporting the involvement of sirtuins in CR-mediated effect on survival is quite strong. CR stimulates the activity of several sirtuins and overexpression of sirtuins increases longevity [88]. Knocking down any one sirtuin gene abolishes the CR-mediated phenotype, including the effect on lifespan.
SIRTs are a family of NAD +− dependent protein deacetylases with pleiotropic intracellular effects, ranging from control of metabolic homeostasis, to limitation of cellular damage, to inhibition of inflammation. The need of NAD+ for enzymatic activity links sirtuins to the energetic status of the cell, with their activity increasing when energy availability is low, i.e. under caloric restriction.
Seven mammalian orthologues (Sirt 1–7) with different subcellular localization have been described so far [89].
The most widely studied is Sirt1, a master regulator of mitochondrial integrity and metabolic homeostasis, localized in the nucleus and cytoplasm of the cell. In the heart, overexpression of Sirt1 attenuates age-dependent cardiac hypertrophy, apoptosis and fibrosis, and expression of senescence markers, in a dose-dependent manner. Moreover, Sirt1 protects the heart from oxidative stress by increasing the expression of antioxidants and decreasing the production of pro-apoptotic molecules [90, 91].
Overexpression of Sirt1 in endothelial cells prevents the appearance of the ROS-mediated senescence phenotype [92]. Sirt1 potentiates eNOS activity, through deacetylation of the enzyme, increasing endothelial nitric oxide availability [93]. Moreover, Sirt1 attenuates vascular NFkB induction, protecting endothelial cells and macrophages from pro-atherosclerotic modifications [94]. Pharmacological activation of Sirt1 reverts the senescent endothelial cell phenotype [95], whereas downregulation of Sirt1 accelerates endothelial senescence [96, 97]. Finally, Sirt1 promotes angiogenesis, through the inhibition of notch signaling pathway in endothelial cells [98].
Sirt3 may also play a role in cardiovascular aging. Sirt3 regulates mitochondrial lysine acetylation, enhancing the antioxidant defense of the cell and preserving mitochondrial function [99]. Overexpression of Sirt3 decreases cellular levels of ROS through deacetylation and activation of the ROS detoxifying enzyme, superoxide dismutase. Sirt3 was reported to prevent stress-induced mitochondrial apoptosis of mammalian cardiomyocytes, and to protect endothelial cells from oxidative damage [100, 101]. Sirt3 deficient mice show cardiac hypertrophy and interstitial fibrosis, whereas Sirt3-overexpressing mice are protected from age-related cardiac modifications [102].
Sirt6 is a nuclear chromatin-associated deacetylase with a critical role in telomere stabilization [103]. The heart has a high expression of Sirt6, suggesting a possible involvement of this protein in myocardial physiology [104]. Cardiomyocytes overexpressing Sirt6 are protected from hypoxic injury, an effect that has been attributed, at least in part, to Sirt6-mediated inactivation of NFkB [105].
The ability of Sirt6 to interfere with NFkB intracellular signaling is of particular importance in vascular biology, where inflammation is the key regulator of atherogenesis. Sirt6 deficient endothelial cells display an increased expression of pro-inflammatory cytokines, such as interleukin 1β and NFkB, in vitro [106].
Sirt7 is the only sirtuin mainly localized in the nucleoli. Very little is known about Sirt7 biological activities and intracellular targets. However, there is evidence indicating that Sirt7 plays a major protective role in the adult myocardium. Sirt7-deficient mice develop progressive heart hypertrophy, accompanied by cardiomyocytes inflammation and decreased stress resistance, and die of inflammatory cardiomyopathy related to a multi-systemic mitochondrial dysfunction [107].
A fair amount of research is devoted to identify compounds mimicking CR positive effects, without the need to reduce calorie intake. Among them, the phytochemicals resveratrol and quercetin, and the drug rapamycin, are the most widely studied [108, 109]. Resveratrol has been shown to inhibits induction of apoptosis by oxidative stress in cultured rat arteries [110] and inflammatory gene expression in human endothelium [111], providing the first concrete pharmacological alternative to CR to preserve a healthier cardiovascular system.
3.4 Epigenetic Regulation and Cardiovascular Aging
Epigenetic changes are considered a major hallmark of aging [112]. The majority of age-related modifications observed in elderly organisms can be explained by changes in tissue-specific epigenetic background. It is widely accepted that the epigenetic trait contributes to the cardiovascular phenotype of an individual and to the pathogenesis of cardiovascular diseases.
The “epigenetic trait” may be defined as a “stably heritable phenotype resulting from changes in the chromosome without alterations in the DNA sequence” [113]. These traits are the result of modification in gene expression, regulated by changes in chromatin structure.
Epigenetic changes may be influenced by exogenous factors, explaining how the environment can shape the genome in defining the phenotype of an individual (gene-environment interaction) [114].
Three major types of epigenetic modifications play a role in shaping DNA accessibility by transcription factors: DNA methylation, histone post-translational modification and noncoding RNAs. However, many other mechanisms of chromatin rearrangement may exist that we still don’t know or completely understand that interact with the major epigenetic modifications in shaping the genome. These epigenetic mechanisms confer to the cell an extraordinary ability to react to physiologic and pathologic stimuli.
DNA Methylation and Demethylation
DNA methylation is a covalent modification consisting in the addition of a methyl group to the carbon 5 of a cytosine residue. In adult somatic cells, DNA methylation occurs typically in the context of a CpG dinucleotide. Methylation of DNA changes its biophysical characteristics, inhibiting the recognition of DNA by some transcription factors or allowing the binding of transcriptional repressors, impacting the overall gene transcription [115]. Together with DNA methylation, demethylation occurs but this mechanism is less understood. Demethylation plays a crucial role in modulating transcriptional responses to hormones and other stimuli, and global loss of methylation has been associated with several pathological processes, including cardiovascular diseases [116].
A study on 421 individuals (age 14–94) demonstrated that the human “methylome” is deeply modified by age [117]. Centenarians confirmed this finding revealing that their methylome exhibits dramatic changes compared to that of young subjects [118]. This gradual change in the genome methylation profile over time is defined as “DNA methylation drift”.
Indeed, several evidence indicates that the overall DNA methylation of mammalian genome decreases with age. Interestingly, in a recent study on frailty, the global DNA methylation level observed in old frail individuals was lower compared to prefrail and nonfrail subjects, suggesting that relaxation of the epigenetic control may be associated with the functional decline of old age [119]. The genome hypomethylation here described could exert pro-ageing effects by promoting genomic instability [120].
However, the age-associated methylome modification is exquisitely complex. In parallel to global hypomethylation, aging seems associated with a trend towards an increase of DNA methylation for specific genomic loci.
Although the epigenomic landscape varies widely across different tissue types, recent studies have identified an age-dependent CpG signature that can predict age across a wide range of tissues and cell types [121]. This CpG signature, referred to as DNAm age, is based on 353 CpGs which, as a whole, identify an “epigenetic clock”. Studies are ongoing to determine the correlation between DNAm age, aging and cardiovascular disease.
Histone Modifications
Histones undergo a variety of post-translational modifications, including acetylation, methylation, phosphorylation, and ubiquitination, that change the chromatin structure and the gene expression profile of the cell. Different types of histone post-translational modifications induce different transcriptional profiles.
Noncoding RNA and RNA Epigenetics
Noncoding RNAs can silence genes by recruitment of remodeling complexes that promote histone methylation. They can also recruit RNA-binding proteins, impairing histone deacetylation or inhibiting transcription factor binding to promoter regions. Although less understood, methylation and demethylation of RNAs (tRNA, mRNA, and rRNA) also occur inside the cell and represent another type of epigenetics [114].
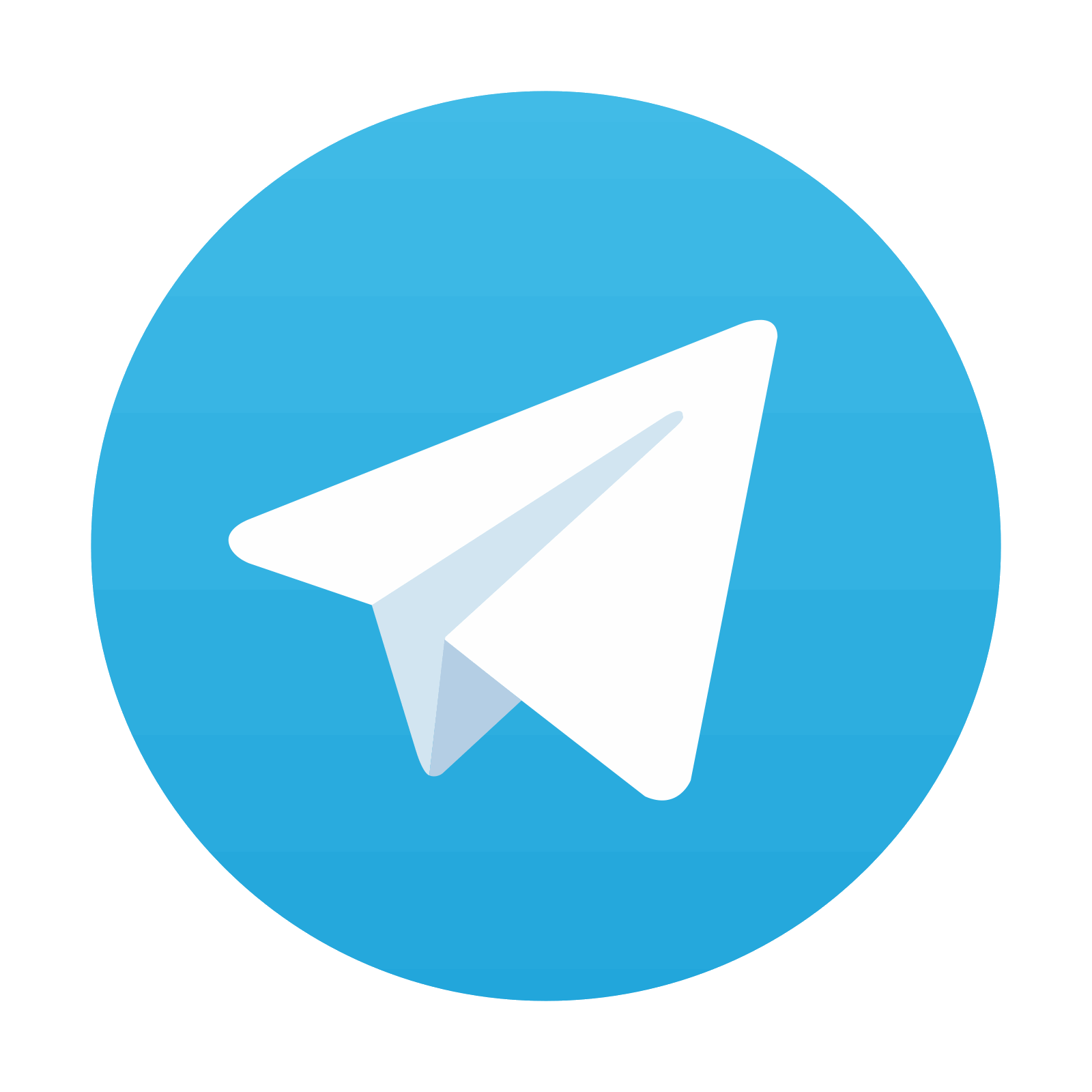
Stay updated, free articles. Join our Telegram channel
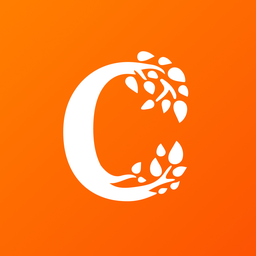
Full access? Get Clinical Tree
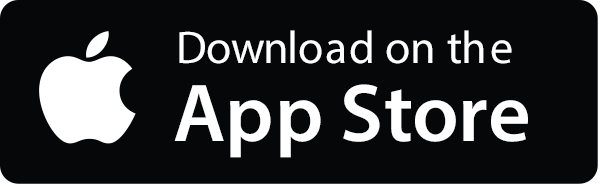
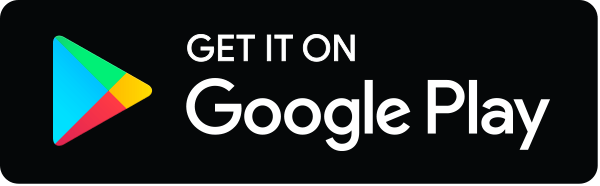