Abstract
Cardiomyocytes maintain a difference in the concentration of ions across their membranes, resulting in a difference in the electrical charge (i.e., voltage difference) across the cell membrane, called the membrane potential. During physiological electrical activity, the membrane potential is a continuous function of time. The current flowing through the cell membrane, at each instant, is provided by multiple channels and transporters carrying charge in opposite directions because of their different ion selectivity.
The cardiac action potential reflects a balance between inward and outward currents. When a depolarizing stimulus abruptly changes the resting membrane potential to a critical value (the threshold level), the properties of the cell membrane and ion conductances change dramatically, precipitating a sequence of events involving the influx and efflux of multiple ions that together produce the action potential of the cell. The course of the action potential can be divided into five phases (numbered 0 to 4). Substantial differences in expression levels of ion channels underlie the considerable heterogeneity in action potential duration and configuration between cardiomyocytes located in different regions of the heart.
Cardiac excitation involves the propagation of the electrical impulse along the cardiac muscle fiber and rapidly from cell to cell. Conduction velocity refers to the speed of propagation of an electrical impulse through cardiac tissue, which is dependent on both the active membrane properties of the individual cardiac myocyte (i.e., electrical excitability and refractoriness) and passive properties governing the flow of current between cardiac cells (cell-to-cell coupling and tissue geometry).
Keywords
cardiac action potential, membrane potential, ion channels, excitability, electrical propagation, refractoriness, excitation-contraction coupling
Outline
Ionic Equilibrium, 1
Transmembrane Potentials, 1
Cardiac Action Potential, 2
Excitability, 8
Refractoriness, 9
Propagation, 10
Intracellular Propagation, 10
Intercellular Propagation, 10
Anisotropic Conduction, 11
Source-Sink Relationship, 11
Safety Factor for Conduction, 12
Excitation-Contraction Coupling, 12
Ionic Equilibrium
The lipid bilayer of the cell membrane is hydrophobic and impermeable to water-soluble substances such as ions. Hence, for the hydrophilic ions to be able to cross the membrane, they need hydrophilic paths that span the membrane (i.e., pores), which are provided by transmembrane proteins called ion channels. Once a hydrophilic pore is available, ions move passively across the membrane, driven by two forces: the electrical gradient (voltage difference) and chemical gradient (concentration difference). The chemical gradient forces the ions to move from a compartment of a higher concentration to one of lower concentration. The electrical gradient forces ions to move in the direction of their inverse sign (i.e., cations [positively charged ions] move toward a negatively charged compartment, whereas anions [negatively charged ions] move toward a positively charged compartment). Because the chemical and electrical gradients can oppose each other, the direction of net ion movement will depend on the relative contributions of chemical gradient and electrical potential (i.e., the net electrochemical gradient), so that ions tend to move spontaneously from a higher to a lower electrochemical potential.
The movement of an ion down its chemical gradient in one direction across the cell membrane results in build-up of excess charge carried by the ion on one side of the membrane, which generates an electrical gradient that impedes (repels) continuing ionic movement in the same direction. When the driving force of the electrical gradient across the membrane becomes equal and opposite to the force generated by the chemical gradient, the ion is said to be in electrochemical equilibrium, and the net transmembrane flux (or current) of that particular ion is zero. In this setting, the membrane electrical potential is called the equilibrium potential (E ion ) (“reversal potential” or “Nernst potential”) of that individual ion. Any further current flow would reverse the balance of forces and therefore reverse the current direction until equilibrium is restored, hence the name “reversal potential.” The E ion for a given ion measures the voltage that the ion concentration gradient generates when it acts as a battery, and it depends on its concentration on either side of the membrane and the temperature. At membrane voltages more positive to the reversal potential of the ion, passive ion movement is outward, whereas it is inward at a membrane potential (E m ) more negative to the Nernst potential of that channel.
When multiple ions across a membrane are removed from their electrochemical equilibrium, each ion will tend to force the E m toward its own E ion . The contribution of each ion type to the overall E m at any given moment is determined by the instantaneous permeability of the plasma membrane to that ion. The larger the membrane conductance to a particular ion, the greater is the ability of that ion to bring the E m toward its own E ion . Hence the E m is the average of the E ion of all the ions to which the membrane is permeable, weighed according to the membrane conductance of each individual ion relative to the total ionic conductance of the membrane.
Transmembrane Potentials
All living cells, including cardiomyocytes, maintain a difference in the concentration of ions across their membranes. There is a slight excess of positive ions on the outside of the membrane and a slight excess of negative ions on the inside of the membrane, resulting in a difference in the electrical charge (i.e., voltage, potential difference, or electrical gradient) across the cell membrane, called the E m (also known as membrane voltage or transmembrane potential). A membrane that exhibits an electrical gradient is said to be polarized.
In nonexcitable cells, and in excitable cells in their baseline states (i.e., not conducting electrical signals), the E m is held at a relatively stable value, called the resting E m . All cells have a negative resting E m (i.e., the cytoplasm is electrically negative relative to the extracellular fluid), which arises from the interaction of ion channels and ion pumps embedded in the membrane that maintain different ion concentrations on the intracellular and extracellular sides of the membrane.
When an ion channel opens, it allows ion flux across the membrane that generates an electrical current (I). This current affects the E m , depending on the membrane resistance (R), which refers to the ratio between the E m and electrical current, as shown in Ohm’s law: E = I × R, or R = E/I. Resistance arises from the fact that the membrane impedes the movement of charges across it; hence the cell membrane functions as a resistor (i.e., when current is passed through the membrane, there is a voltage drop that is predictable from Ohm’s law). Conductance describes the ability of a membrane to allow the flux of charged ions in one direction across the membrane. The more permeable the membrane is to a particular ion, the greater is the conductance of the membrane to that ion ( Table 1.1 ). Membrane conductance (g) is the reciprocal of resistance: g = 1/R.
Term | Unit | Definition |
---|---|---|
Charge (electric charge, Q) | Coulombs |
|
Voltage (potential difference, V) | Volt (V) |
|
Current (I) | Amperes (A) |
|
Resistance (R) | Ohm (Ω) |
|
Conductance (g) | Siemen (S) |
|
Capacitance (C) | Farad |
|
Membrane potential (transmembrane potential, membrane voltage, E m ) | Volt (V) |
|
Equilibrium potential of an ion (E ion ) (reversal potential, Nernst potential) | Volt (V) |
|
Ionic current (I ion ) | Amperes (A) |
|
Capacitive current (nonfaradaic current, double-layer current) |
|
Because the lipid bilayer of the cell membrane is very thin, accumulation of charged ions on one side gives rise to an electrical force (potential) that pulls oppositely charged particles toward the other side. Hence the cell membrane functions as a capacitor (i.e., capable of separating and storing charge). Although the absolute potential differences across the cell membrane are small, they give rise to enormous electrical potential gradients because they occur across a very thin surface. As a consequence, apparently small changes in E m can produce large changes in potential gradient and powerful forces that are able to induce molecular rearrangement in membrane proteins, such as those required for opening and closing ion channels embedded in the cell membrane. The capacitance of the membrane is generally fixed and unaffected by the molecules that are embedded in it. In contrast, membrane resistance is highly variable and depends on the conductance of ion channels embedded in the membrane.
The sodium (Na + ), potassium (K + ), calcium (Ca 2+ ), and chloride (Cl − ) ions are the major charge carriers, and their movement across the cell membrane creates a flow of current that generates excitation and signals in cardiac myocytes. The electrical current generated by the flux of an ion across the membrane is determined by the membrane conductance to that ion (g ion ) and the potential (voltage) difference across the membrane. The potential difference represents the potential at which there is no net ion flux (i.e., the E ion ) and the actual E m : current = g ion × (E m − E ion ).
By convention, an inward current increases the electropositivity within the cell (i.e., causes depolarization of the E m [to become less negative]) and can result from either the movement of positively charged ions (most commonly Na + or Ca 2+ ) into the cell or the efflux of negatively charged ions (e.g., Cl − ) out of the cell. An outward current increases the electronegativity within the cell (i.e., causes hyperpolarization of the E m [to become more negative]) and can result from either the movement of anions into the cell or the efflux of cations (most commonly K + ) out of the cell.
Opening and closing of ion channels can induce a departure from the relatively static resting E m , which is called depolarization if the interior voltage rises (becomes less negative) or hyperpolarization if the interior voltage becomes more negative. The most important ion fluxes that depolarize or repolarize the membrane are passive (i.e., the ions move down their electrochemical gradient without requiring the expenditure of energy), occurring through transmembrane ion channels. In excitable cells a sufficiently large depolarization can evoke a short-lasting all-or-none event called an action potential, in which the E m very rapidly undergoes specific and large dynamic voltage changes.
Both resting E m and dynamic voltage changes such as the action potential are caused by specific changes in membrane permeabilities for Na + , K + , Ca 2+ , and Cl − , which, in turn, result from concerted changes in functional activity of various ion channels, ion transporters, and ion exchangers.
Cardiac Action Potential
During physiological electrical activity, the E m is a continuous function of time. The current flowing through the cell membrane, at each instant, is provided by multiple channels and transporters carrying charge in opposite directions because of their different ion selectivity. The algebraic summation of these contributions is referred to as net transmembrane current.
The cardiac action potential reflects a balance between inward and outward currents. When a depolarizing stimulus (typically generated by an electric current from an adjacent cell) abruptly changes the E m of a resting cardiomyocyte to a critical value (the threshold level), the properties of the cell membrane and ion conductances change dramatically, precipitating a sequence of events involving the influx and efflux of multiple ions that together produce the action potential of the cell. In this fashion an electrical stimulus is conducted from one cell to the cells adjacent to it.
Unlike skeletal muscle, cardiac muscle is electrically coupled so that the wave of depolarization propagates from one cell to the next, independent of neuronal input. The heart is activated by capacitive currents generated when a wave of depolarization approaches a region of the heart that is at its resting potential. Unlike ionic currents, which are generated by the flux of charged ions across the cell membrane, capacitive currents are generated by the movement of electrons toward and away from the surfaces of the membrane. These electrotonic potential changes are passive and independent of membrane conductance. The resulting decrease in positive charge at the outer side of the cell membrane reduces the negative charge on the intracellular surface of the membrane. These charge movements, which are carried by electrons, generate a capacitive current. When an excitatory stimulus causes the E m to become less negative and beyond a threshold level (approximately −65 mV for working atrial and ventricular cardiomyocytes), Na + channels activate (open) and permit an inward Na + current (I Na ), resulting in a rapid shift of the E m to a positive voltage range. This event triggers a series of successive opening and closure of selectively permeable ion channels. The direction and magnitude of passive movement (and the resulting current) of an ion at any given transmembrane voltage are determined by the ratio of the intracellular and extracellular concentrations and the reversal potential of that ion, with the net flux being larger when ions move from the more concentrated side.
The “threshold potential” is the lowest E m at which opening of enough Na + channels (or Ca 2+ channels in the setting of nodal cells) is able to initiate the sequence of channel openings needed to generate a propagated action potential. Small (subthreshold) stimuli depolarize the membrane in proportion to the strength of the stimulus and cause only local responses because they do not open enough Na + channels to generate depolarizing currents large enough to activate nearby resting cells (i.e., insufficient to initiate a regenerative action potential). On the other hand, when the stimulus is sufficiently intense to reduce the E m to a threshold value, regenerative action potential results, whereby intracellular movement of Na + depolarizes the membrane more, a process that increases conductance to Na + more, which allows more Na + to enter, and so on. In this fashion the extent of subsequent depolarization becomes independent of the initial depolarizing stimulus, and more intense stimuli do not produce larger action potential responses; rather, an all-or-none response results.
Electrical changes in the action potential follow a relatively fixed time and voltage relationship that differs according to specific cell types. Although the entire action potential takes only a few milliseconds in nerve cells, the cardiac action potential lasts several hundred milliseconds. The course of the action potential can be divided into five phases (numbered 0 to 4). Phase 4 is the resting E m , and it describes the E m when the cell is not being stimulated.
During the cardiac action potential, membrane voltages fluctuate in the range of −94 to +30 mV ( Fig. 1.1 ). With physiological external K + concentration, the reversal potential of K + (E K ) is approximately −94 mV, and passive K + movement during an action potential is out of the cell. On the other hand, because the calculated reversal potential of a cardiac Ca 2+ channel (E Ca ) is +64 mV, passive Ca 2+ flux is into the cell.

In normal atrial and ventricular myocytes and in His-Purkinje fibers, action potentials have very rapid upstrokes, mediated by the fast inward I Na . These potentials are called fast response potentials. In contrast, action potentials in the normal sinus and atrioventricular (AV) nodal cells and many types of diseased tissues have very slow upstrokes, mediated by a slow inward, predominantly L-type voltage-gated Ca 2+ current (I CaL ), rather than by the fast inward I Na ( Fig. 1.2 ). These potentials have been termed slow response potentials.

Fast Response Action Potential
Phase 4: The Resting Membrane Potential
The E m of resting atrial and ventricular cardiomyocytes remains steady throughout diastole. The resting E m is caused by the differences in ionic concentrations across the membrane and the selective membrane permeability (conductance) to various ions. Large concentration gradients of Na + , K + , Ca 2+ , and Cl − across the cell membrane are maintained by the ion pumps and exchangers ( Table 1.2 ).
Ion | Extracellular Concentration (mM) | Intracellular Concentration (mM) | Equilibrium Potential (mV) |
---|---|---|---|
Na + | 135–145 | 10 | +70 |
K + | 3.5–5.0 | 155 | −94 |
Ca 2+ | 2 | 0.0001 | +132 |
Cl − | 87 | 30 | −28 |
Under normal conditions, the resting membrane is most permeable to K + and relatively impermeable to other ions. K + has the largest resting membrane conductance (g K is 100 times greater than g Na ) because of the abundance of open K + channels at rest, whereas Na + and Ca 2+ channels are generally closed. Thus K + exerts the largest influence on the resting E m . As a consequence, the resulting E m is almost always close to the K + reversal potential (E m approximates E K ). The actual resting E m is slightly less negative than E K because the cell membrane is slightly permeable to other ions.
The inwardly rectifying K + (Kir) channels underlie an outward K + current (I K1 ) responsible for maintaining the resting potential near the E K in atrial, His-Purkinje, and ventricular cells, under normal conditions. Kir channels preferentially allow currents of K + ions to flow into the cell with a strongly voltage-dependent decline of K + efflux (i.e., reduction of outward current) on membrane depolarization. As such, I K1 is a strong rectifier that passes K + currents over a limited range of E m . At a negative E m , I K1 conductance is much larger than that of any other current; thus it clamps the resting E m close to the reversal potential for K + (E K ) (see Chapter 2 for detailed discussion on the concept of rectification). I K1 density is much higher in ventricular than in atrial myocytes, a feature that largely prevents the ventricular cell from having diastolic membrane depolarization and pacemaker activity. By contrast, I K1 is almost absent in sinus and AV nodal cells, thus allowing for relatively more depolarized resting diastolic potentials compared with atrial and ventricular myocytes ( Table 1.3 ). The effect of outward K + current to resist membrane depolarization (keeping voltage fixed) is sometimes referred to as a voltage clamping effect.
Property | Sinus Nodal Cell | Atrial Muscle Cell | AV Nodal Cell | Purkinje Fiber | Ventricular Muscle Cell |
---|---|---|---|---|---|
Resting potential (mV) | −50 to −60 | −80 to −90 | −60 to −70 | −90 to −95 | −80 to −90 |
Action potential amplitude (mV) | +60 to +70 | +110 to +120 | +70 to +80 | +120 | +110 to +120 |
Action potential duration (msec) | 100 to 300 | 100 to 300 | 100 to 300 | 300 to 500 | 200 to 300 |
A unique property of Kir currents is the unusual dependence of rectification on extracellular K + concentration. Specifically, with an increase in extracellular K + , the I K1 current-voltage relationship shifts nearly in parallel with the E K and leads to a crossover phenomenon. One important consequence of such behavior is that at potentials positive to the crossover, K + conductance increases rather than decreases, against an expectation based on a reduced driving force for K + ions as a result of elevated extracellular K + concentration.
The resting E m is also powered by the Na + -K + adenosine triphosphatase (ATPase) (the Na + -K + pump), which helps to establish concentration gradients of Na + and K + across the cell membrane. Under physiological conditions, the Na + -K + pump transports two K + ions into the cell against its chemical gradient and three Na + ions outside against its electrochemical gradient at the expense of one ATP molecule. Because the stoichiometry of ion movement is not 1 : 1, the Na + -K + pump is electrogenic and generates a net outward movement of positive charges (i.e., an outward current). At faster heart rates, the rate of Na + -K + pumping increases to maintain the same ionic gradients, thus counteracting the intracellular gain of Na + and loss of K + with each depolarization.
Ca 2+ does not contribute directly to the resting E m because the voltage-activated Ca 2+ channels are closed at the hyperpolarized resting E m . However, changes in intracellular free Ca 2+ concentration can affect other membrane conductance values. Increases in intracellular Ca 2+ levels can stimulate the Na + -Ca 2+ exchanger (I Na-Ca ), which exchanges three Na + ions for one Ca 2+ ion; the direction depends on the Na + and Ca 2+ concentrations on the two sides of the membrane and the E m difference. At resting E m and during a spontaneous sarcoplasmic reticulum Ca 2+ release event, this exchanger would generate a net Na + influx, possibly causing transient membrane depolarizations.
Phase 0: The Upstroke—Rapid Depolarization
On excitation of an atrial, ventricular, or Purkinje cardiomyocyte by electrical stimuli from adjacent cells, its resting E m (approximately −85 mV) depolarizes, leading to opening (activation) of Na + channels from its resting (closed) state and enabling a large and rapid influx of Na + ions (inward I Na ) into the cell down their electrochemical gradient. As a consequence of increased Na + conductance, the excited membrane no longer behaves like a K + electrode (i.e., exclusively permeable to K + ) but more closely approximates an Na + electrode, and the E m moves toward the E Na (see Table 1.2 ). Once an excitatory stimulus depolarizes the E m beyond the threshold for activation of Na + channels (approximately −65 mV), the activated I Na is regenerative and no longer depends on the initial depolarizing stimulus. As a consequence, the influx of Na + ions further depolarizes the membrane and thereby increases conductance to Na + more, which allows more Na + to enter the cell (thus “regenerative”).
Normally, activation of Na + channels is transient; fast inactivation (closing of the channel pore) starts simultaneously with activation, but because inactivation is slightly delayed relative to activation, the channels remain transiently (less than 1 millisecond) open to conduct I Na during phase 0 of the action potential before it closes. In addition, the influx of Na + into the cell increases the positive intracellular charges and reduces the driving force for Na + . When the E Na is reached, no further Na + ions enter the cell.
The rate at which depolarization occurs during phase 0 (i.e., the maximum rate of change of voltage over time [dV/dt max ]) is a reasonable approximation of the rate and magnitude of Na + entry into the cell and a determinant of conduction velocity for the propagated action potential (see later).
The threshold for activation of I CaL is approximately −30 to −40 mV. Although I CaL is normally activated during phase 0 by the regenerative depolarization caused by the fast I Na , I CaL is much smaller than the peak I Na . Furthermore, the amplitude of I CaL is not maximal near the action potential peak because of the time-dependent nature of I CaL activation, as well as the low driving force (E m − E Ca ) for I CaL . Therefore I CaL contributes little to the action potential until the fast I Na is inactivated, after completion of phase 0. As a result, I CaL affects mainly the plateau of action potentials recorded in atrial and ventricular muscle and His-Purkinje fibers. On the other hand, I CaL plays a prominent role in the upstroke of slow response action potentials in partially depolarized cells in which the fast Na + channels have been inactivated.
Phase 1: Early Repolarization
Phase 0 is followed by phase 1 (early repolarization), during which the membrane repolarizes rapidly and transiently to almost 0 mV (early notch), partly because of the inactivation of I Na and concomitant activation of several outward currents. The transient outward K + current (I to ) is mainly responsible for phase 1 of the action potential. I to rapidly activates (with time constants less than 10 milliseconds) by depolarization and then rapidly inactivates (25 to 80 milliseconds for the fast component of I to [I to,f ], and 80 to 200 milliseconds for the slow component of I to [I to,s ]). The influx of K + ions via I to channels partially repolarizes the membrane, thus shaping the rapid repolarization (phase 1) of the action potential and setting the height of the initial plateau (phase 2) (see Fig. 1.1 ). In addition, an Na + outward current through the Na + -Ca 2+ exchanger operating in reverse mode likely contributes to this early phase of repolarization.
Phase 2: The Plateau
Phase 2 (plateau) represents a delicate balance between the depolarizing inward currents (I CaL and a small residual component of inward I Na ) and the repolarizing outward currents (ultrarapidly [I Kur ], rapidly [I Kr ], and slowly [I Ks ] activating delayed outward rectifying currents) (see Fig. 1.1 ). Phase 2 is the longest phase of the action potential, lasting tens (atrium) to hundreds of milliseconds (His-Purkinje system and ventricle). The plateau phase is unique among excitable cells and marks the phase of Ca 2+ entry into the cell. It is the phase that most clearly distinguishes the cardiac action potential from the brief action potentials of skeletal muscle and nerve.
I CaL is activated by membrane depolarization, is largely responsible for the action potential plateau, and is a major determinant of the duration of the plateau phase. I CaL also links membrane depolarization to myocardial contraction. L-type Ca 2+ channels activate on membrane depolarization to potentials positive to −40 mV. I CaL peaks at an E m of 0 to +10 mV and tends to reverse at +60 to +70 mV, following a bell-shaped current-voltage relationship.
Na + channels also make a contribution, although minor, to the plateau phase. After phase 0 of the action potential, some Na + channels occasionally fail to inactivate or exhibit prolonged opening or reopening repetitively for hundreds of milliseconds after variable and prolonged latencies, resulting in a small inward I Na (with a magnitude of less than 1% of the peak I Na ). This persistent or “late” I Na (I NaL ), along with I CaL , helps to maintain the action potential plateau.
I Kr and I Ks are activated at depolarized membrane potentials. I Kr activates relatively fast (in the order of tens of milliseconds) on membrane depolarization, thus allowing outward diffusion of K + ions in accordance with its electrochemical gradient, but voltage-dependent inactivation thereafter is very fast. Hence only limited numbers of channels remain in the open state, whereas a considerable fraction resides in the nonconducting inactivated state. The fast voltage-dependent inactivation limits outward current through the channel at positive voltages and thus helps to maintain the action potential plateau phase that controls contraction and prevents premature excitation. However, as the voltage becomes less positive at the end of the plateau phase of repolarization, the channels recover rapidly from inactivation; this process leads to a progressive increase in I Kr amplitudes during action potential phases 2 and 3, with maximal outward current occurring before the final rapid declining phase of the action potential.
I Ks , which is approximately 10 times larger than I Kr , also contributes to the plateau phase. I Ks activates in response to membrane depolarization to potentials positive to −30 mV and gradually increases during the plateau phase because its time course of activation is extremely slow, slower than any other known K + current. In fact, steady-state amplitude of I Ks is achieved only with extremely long membrane depolarization. Hence the contribution of I Ks to the net repolarizing current is greatest late in the plateau phase, particularly during action potentials of long duration. Importantly, although I Ks activates slowly compared with action potential duration, it is also slowly inactivated. As heart rate increases, I Ks increases because channel deactivation is slow and incomplete during the shortened diastole. This allows I Ks channels to accumulate in the open state during rapid successive action potentials and mediate the faster rate of repolarization. Hence I Ks plays an important role in determining the rate-dependent shortening of the cardiac action potential.
I Kur is detected only in human atria but not in the ventricles, such that it is the predominant delayed rectifier current responsible for atrial repolarization and is a basis for the much shorter duration of the action potential in the atrium. I Kur activates rapidly on depolarization in the plateau range and displays outward rectification, but it inactivates slowly during the time course of the action potential.
The Na + -Ca 2+ exchanger operating in forward mode (three Na + ions in for one Ca 2+ ion out) and the Na + -K + pump provide minor current components during phase 2.
Importantly, during the plateau phase, membrane conductance to all ions falls to rather low values. Thus less change in current is required near plateau levels than near resting potential levels to produce the same changes in E m . In particular, K + conductance falls during the plateau phase as a result of inward rectification of I K1 (i.e., voltage-dependent decline of K + efflux and hence reduction of outward current) on membrane depolarization, in spite of the large electrochemical driving force on K + ions during the positive phase of the action potential (phases 0, 1, and 2). This property allows membrane depolarization following Na + channel activation, slows membrane repolarization, and helps to maintain a more prolonged cardiac action potential. This also confers energetic efficiency in the generation of the action potential.
Phase 3: Final Rapid Repolarization
Phase 3 is the phase of rapid repolarization that restores the E m to its resting value. Phase 3 is mediated by the increasing conductance of the delayed outward rectifying currents (I Kr and I Ks ), the inwardly rectifying K + currents (I K1 and acetylcholine-activated K + current [I KACh ]), and time-dependent inactivation of I CaL (see Fig. 1.1 ). Final repolarization during phase 3 results from K + efflux through the I K1 channels, which open at potentials negative to −20 mV.
Phase 4: Restoration of Resting Membrane Potential
During the action potential, Na + and Ca 2+ ions enter the cell and depolarize the E m . Although the E m is quickly repolarized by the efflux of K + ions, restoration of transmembrane ionic concentration gradients to the baseline resting state is necessary. This is achieved by the Na + -K + ATPase (Na + -K + pump, which exchanges two K + ions inside and three Na + ions outside) and by the Na + -Ca 2+ exchanger (I Na-Ca , which exchanges three Na + ions for one Ca 2+ ion).
Reduction of cytosolic Ca 2+ concentration during diastole is achieved by the reuptake Ca 2+ by the sarcoplasmic reticulum via activation of the sarco/endoplasmic reticulum calcium-ATPase calcium pump (SERCA), in addition to extrusion across the sarcolemma via the Na + -Ca 2+ exchanger. In the human heart under resting conditions, the time required for cardiac myocyte depolarization, contraction, relaxation, and recovery is approximately 600 milliseconds.
Regional Heterogeneity of the Action Potential
Substantial differences in expression levels of ion channels underlie the considerable heterogeneity in action potential duration and configuration between cardiomyocytes located in different regions of the heart. The characteristics of the action potential differ in atrial versus ventricular myocardium, as well as across the ventricular myocardial wall from endocardium, midmyocardium, to epicardium (see Fig. 1.2 ).
Atrioventricular Heterogeneity of the Action Potential
Compared with the atrium, ventricular myocytes maintain a slightly more hyperpolarized resting E m (approximately −85 mV vs. −80 mV). In addition, the action potential duration is longer, the plateau phase reaches a more depolarized E m (approximately +20 mV), and phase 3 repolarization curve is steeper in ventricular myocytes as compared with the atrial action potential (see Table 1.3 ).
The differences in action potential configuration between atria and ventricles are mainly related to differences in ionic current densities and ion channel expression (especially K + channels) between ventricular and atrial myocytes. Although I Kr and I Ks densities are similar in atrial and ventricular myocytes, I Kur is detected only in human atria and not in the ventricles. In fact, I Kur is the predominant delayed rectifier current responsible for human atrial repolarization, with only small contribution of I Kr and I Ks .
Furthermore, the density of I to is twofold higher in the atria compared with ventricular myocytes. In addition, I to subtypes (I to,f and I to,s ) are differentially expressed in the heart. I to,f is the principal subtype expressed in human atrium. Conversely, I to,s is larger and I to,f is smaller in the ventricles compared with atrial tissue.
The markedly higher densities of I to,f , together with the expression of I Kur , accelerate the early phase of repolarization and lead to lower plateau potentials and shorter action potential durations in atrial as compared with ventricular cells.
I K1 density is much higher in ventricular than in atrial myocytes, and this explains the steep repolarization phase in the ventricles (where the more abundant I K1 plays a larger role in accelerating the terminal portion of repolarization) and the shallower phase in the atria. Furthermore, the higher I K1 channel expression underlies the hyperpolarized resting E m in ventricular myocytes, and prevents the ventricular cell from exhibiting pacemaker activity.
Several other K + channels are atrial selective and potentially contribute significantly to the atrial, but not ventricular, action potential. These include I KACh , two-pore K + channels (K 2P ), and small-conductance Ca 2+ -activated K + (SK) channels.
Ventricular Regional Heterogeneity of the Action Potential
Action potential differences exist among the different layers across the ventricular wall, between the left and right ventricles, and from the apical region to the base.
Three distinct action potential waveforms have been distinguished from three predominant cell types contributing to the transmural heterogeneity of ventricular repolarization: the epicardial, midmyocardial, and endocardial cardiomyocytes. The most notable differences among these three layers are the prominent phase 1 notch and the spike and dome morphology of epicardial and midmyocardial action potentials compared with endocardium. The action potential duration of epicardial myocytes is shorter than that of endocardial myocytes. The duration of the action potential is longest in midmyocardial myocytes.
The distinct notch phase in the action potential waveform of epicardial myocytes has mainly been attributed to the regional differences in I to density across the myocardial wall. In human ventricles, I to densities are much higher in the epicardium and midmyocardium than in the endocardium. Furthermore, although both I to,f and I to,s are expressed in the ventricle, I to,f is more prominent in the epicardium and midmyocardium than in the endocardium, whereas I to,s is mainly present in the endocardium and Purkinje cells. A prominent I to -mediated action potential notch in ventricular epicardium but not endocardium produces a transmural voltage gradient during early ventricular repolarization that registers as a J wave or J point elevation on the electrocardiogram (ECG).
Some experimental studies in wedge preparations strongly suggest the presence of a subpopulation of cells in the midmyocardium (referred to as the M cells) that exhibits distinct electrophysiological (EP) properties, although the presence of M cells has not been consistently confirmed by intact heart experiments. The putative M cells appear to have the longest action potential duration across the myocardial wall, largely attributed to their weaker I Ks current but stronger late I Na and Na + -Ca 2+ exchanger currents. Hence the M cells have been proposed to underlie the EP basis for transmural ventricular dispersion of repolarization and the T wave on the surface ECG, with the peak of the T wave (in wedge preparations) coinciding with the end of epicardial repolarization and the end of the T wave coinciding with the end of repolarization of the M cells. Although the role of M cells under physiological conditions remains controversial, these cells appear to have a significant role in arrhythmogenesis under a variety of pathological conditions, such as the long QT and Brugada syndromes, secondary to exaggeration of transmural repolarization gradients.
In addition to the transmural action potential gradient that exists across the three layers of myocardium in the left and right ventricles, the right ventricular (RV) action potential duration overall is shorter and the spike and dome morphology is more pronounced compared with that of the left ventricle (LV). These differences have been attributed to higher I to densities in the right than in the left ventricular myocytes.
Evidence also suggests an apicobasal ventricular action potential heterogeneity. Action potential duration appears to be shorter in LV base compared with the apex. Larger I to and I Ks in apical compared with basal myocytes likely underlie those observations.
Slow Response Action Potential
In normal atrial and ventricular myocytes and in the His-Purkinje fibers, action potentials have very rapid upstrokes mediated by the fast inward I Na . These potentials are called fast response potentials. In contrast, action potentials in the normal sinus and AV nodal cells and many types of diseased tissue have very slow upstrokes, mediated predominantly by the slow inward I CaL , rather than by the fast inward I Na (see Fig. 1.2 ). These potentials have been termed slow response potentials .
As noted, action potentials of pacemaker cells in the sinus and AV nodes are significantly different from those in working atrial and ventricular myocardium. Slow response action potentials are characterized by a more depolarized E m at the onset of phase 4 (−50 to −65 mV), slow diastolic depolarization during phase 4, and reduced action potential amplitude. Furthermore, the rate of depolarization in phase 0 is much slower than that in the working myocardial cells, resulting in reduced conduction velocity of the cardiac impulse in the nodal regions (see Table 1.3 ). Cells in the His-Purkinje system can also exhibit phase 4 depolarization under special circumstances (when Na + channels are inactivated by pathological processes).
Phase 4: Diastolic Depolarization
The sinus and AV nodal cells lack the inward rectifier K + current (I K1 ), which acts to stabilize the resting E m in the normal working atrial and ventricular myocardium and Purkinje fibers. Sinus and AV nodal excitable cells exhibit a spontaneous, slow, and progressive decline in the E m during diastole (spontaneous diastolic depolarization or phase 4 depolarization) that underlies normal automaticity and pacemaking function. Once this spontaneous depolarization reaches threshold (approximately −40 mV), a new action potential is generated.
The ionic mechanisms responsible for diastolic depolarization and normal pacemaker activity in the sinus node are still controversial. Originally, a major role was attributed to the decay of the delayed K + conductance (an outward current) activated during the preceding action potential (the I K -decay theory). This model of pacemaker depolarization lost favor upon the discovery of the “funny” current (I f ), sometimes referred to as the pacemaker current. I f is a hyperpolarization-activated inward current that is carried largely by Na + and, to a lesser extent, K + ions. Once activated, I f depolarizes the membrane to a level where the Ca 2+ current activates to initiate an action potential.
Other ionic currents gated by membrane depolarization (i.e., I CaL and T-type Ca 2+ current [I CaT ]), nongated and nonspecific background leak currents, and a current generated by the Na + -Ca 2+ exchanger have also been proposed to be involved in pacemaking. The “membrane clock” (also referred to as the “voltage clock” or “ion channel clock”) refers to the time- and voltage-dependent membrane ion channels underlying pacemaking activity, including the decay of the outward rectifier K + current and the activation of several inward currents (I f , I CaL , I CaT , and I Na ).
Newer evidence suggests that the sarcoplasmic reticulum, a major Ca 2+ store in sinus nodal cells, can function as a physiological clock (the so-called calcium clock) within the cardiac pacemaker cells and has a substantial impact on late diastolic depolarization.
There remains some degree of uncertainty about the relative role of I f versus that of intracellular Ca 2+ cycling in controlling the normal pacemaker cell automaticity. Furthermore, the interactions between the membrane clock and the intracellular calcium clock and cellular mechanisms underlying this internal Ca 2+ clock are not completely elucidated. A further debate has arisen around their individual (or mutual) relevance in mediating the positive and negative chronotropic effects of neurotransmitters. Nevertheless, these interactions are of fundamental importance for understanding the integration of pacemaker mechanisms at the cellular level (see Chapter 3 for detailed discussion on the mechanisms of automaticity and pacemaker activity).
Phase 0: The Upstroke—Slow Depolarization
I K1 is almost absent in sinus and AV nodal cells, thus allowing for relatively more depolarized resting diastolic potentials (−50 to −65 mV) compared with atrial and ventricular myocytes and facilitating diastolic depolarization mediated by the inward currents (e.g., I f ). At the depolarized level of the maximum diastolic potential of pacemaker cells, most Na + channels are inactivated and unavailable for phase 0 depolarization. Consequently, action potential upstroke is mainly achieved by I CaL .
L-type Ca 2+ channels activate on depolarization to potentials positive to −40 mV, and I CaL peaks at 0 to +10 mV. The peak amplitude I CaL is less than 10% that of I Na , and the time required for activation and inactivation of I CaL is approximately an order of magnitude slower than that for I Na . As a consequence, the rate of depolarization in phase 0 (dV/dt) is much slower and the peak amplitude of the action potential is less than that in the working myocardial cells.
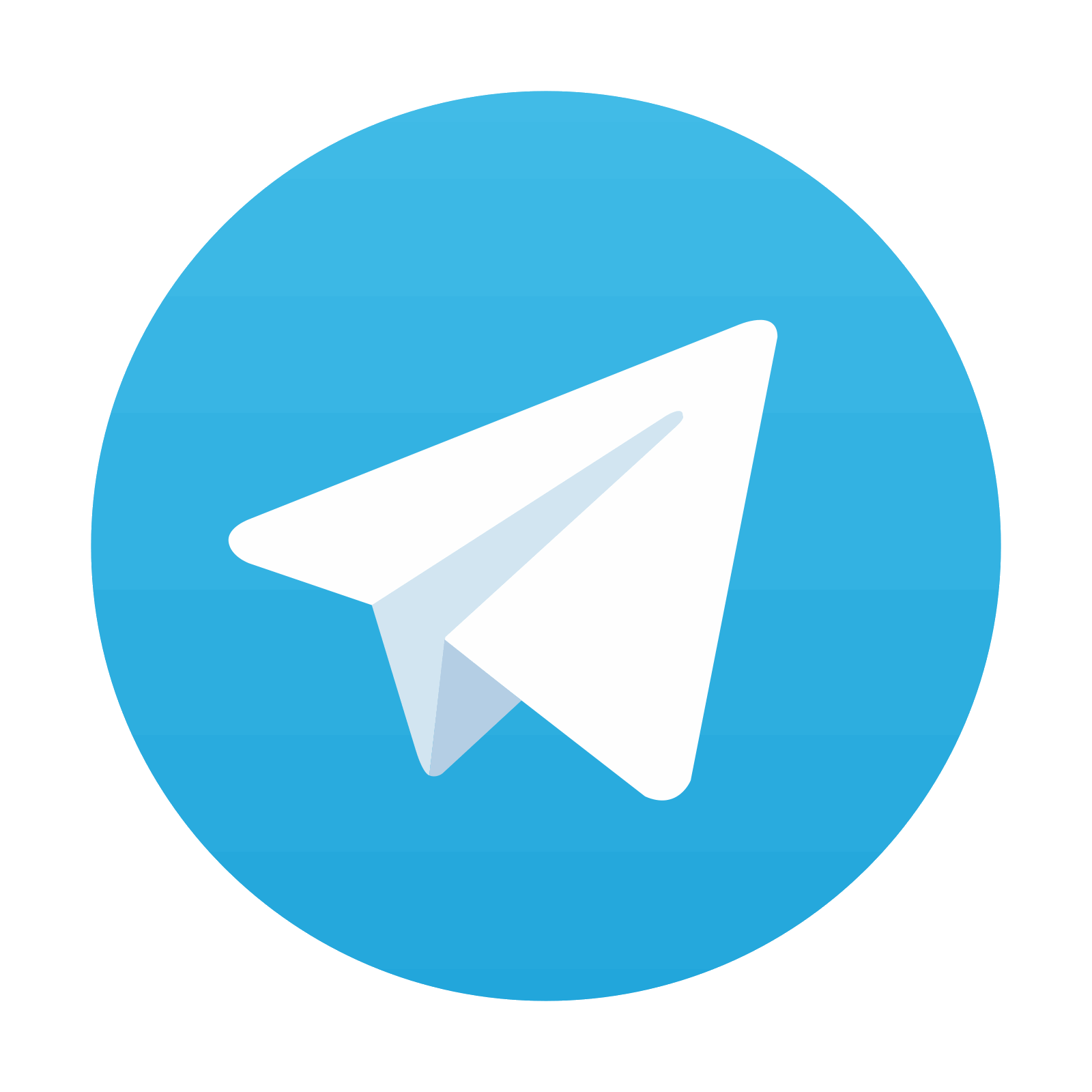
Stay updated, free articles. Join our Telegram channel
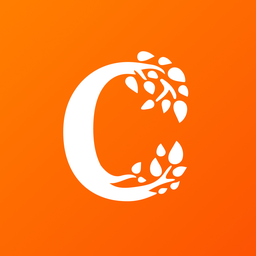
Full access? Get Clinical Tree
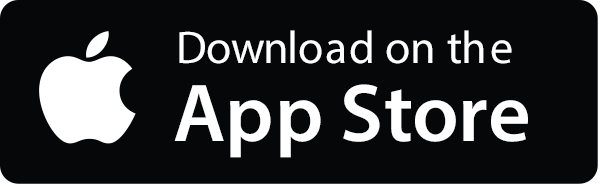
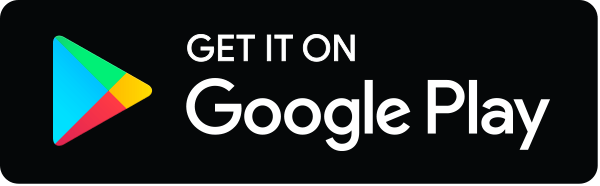