Fig. 10.1
Schematic representation of troponin function during diastole and systole . cTnI (orange), cTnC (blue), and cTnT (cyan) form the troponin complex. In diastole the cTnI inhibitory region (IR), helix 4, and the C-terminal domain (C-TnI) interact with actin to inhibit strong binding sites for myosin on actin. In systole as Ca2+ levels rise, cTnC N-terminal lobe (N-TnC) interacts with Ca2+ which in turn allows cTnI helix 3 (H3) to interact with cTnC. This transition causes cTnI IR and the C-TnI domains to move away from actin allowing tropomyosin to slide on actin revealing myosin strong binding sites
Contractility begins with an action potential, activating the voltage-gated L-type Ca2+ channel to allow a small amount of Ca2+ through the sarcolemma and initiating Ca2+-induced Ca2+ release from the sarcoplasmic reticulum through activation of the ryanodine receptor [6]. This floods the cytosol with Ca2+ where it can bind to the thin filament protein TnC. TnC binding of Ca2+ induces a slight opening of TnC’s hydrophobic pocket, allowing TnI to bind to this region [7]. TnI binding to TnC pulls TnI’s inhibitory region away from actin, enabling Tm to slide on actin, which reveals strong binding sites on actin for myosin [8]. Myosin then strongly binds actin and, in an ATP-dependent manner, produces force. Relaxation initiates through the release of Ca2+ from TnC and subsequent TnI disassociation from TnC and association with Tm/actin, which inhibits myosin strong binding to actin and contraction (◘ Fig. 10.1). Through this process, multiple protein-protein interactions and movements dictate the overall Ca2+ affinity of TnC and force production, referred to as Ca2+ sensitivity [9, 10].
Ca2+ sensitivity of the sarcomere operates in a physiological range defined by normal alterations such as sarcomere length (SL) and posttranslational modifications. On a beat-to-beat basis, SL stretch increases Ca2+ sensitivity, described by the Frank-Starling mechanism, to increase pump function [11]. Phosphorylation of cTnI through a protein kinase A-dependent mechanism decreases Ca2+ sensitivity of the sarcomere [12]. Maintaining Ca2+ sensitivity within a physiologic range is critical to normal heart pump function, and alterations in Ca2+ sensitivity outside the normal operating zone can lead to disease. Myofilament Ca2+ sensitivity is significantly altered in multiple disease states. Myocardial infarction decreases Ca2+ sensitivity through an acidosis-based mechanism [13]. Recently, advanced heart failure has been associated with increased Ca2+ sensitivity, possibly due to decreased cTnI phosphorylation [14]. Mutations within sarcomeric proteins can result in either increased or decreased Ca2+ sensitivity. Either one can lead to disease [15].
Clinical Overview
Sarcomeric cardiomyopathies are defined by a causal mutation in a gene that encodes for a cardiac sarcomeric protein giving rise to a diseased heart [4]. Criteria for a causal mutation usually include: cosegregation with the disease phenotype in a familial manner, prior evidence of pathogenicity, not present in a control population, occurs as an evolutionary conserved residue in a critical portion of the protein and/or major protein structure/function phenotypes in basic science experiments [16]. Genetic mutations in sarcomeric genes have been identified as causative in three different clinically classified cardiomyopathies: (1) dilated cardiomyopathy (DCM), (2) hypertrophic cardiomyopathy (HCM ), and (3) restrictive cardiomyopathy (RCM ) (◘ Fig. 10.2) [8, 17, 18].


Fig. 10.2
Common morphological features of sarcomeric cardiomyopathies in relation to myofilament Ca2+ sensitivity. DCM (pink) hearts have thin left ventricular walls and a dilated left ventricular chamber. HCM hearts show increased thickness of the septal wall and left ventricular free wall. RCM hearts show no hypertrophy but have decreased left ventricular end-diastolic dimensions due to “stiff” walls. Ruler of Ca2+ sensitivity depicting the common molecular phenotype of DCM has decreased Ca2+ sensitivity, while HCM and RCM commonly have increased Ca2+ sensitivity
DCM is clinically characterized by left ventricular dilation and poor systolic function with a high propensity for heart failure, death, or transplant. HCM, on the other hand, is clinically characterized by left ventricular hypertrophy, normal or enhanced systolic function, and impaired left ventricular relaxation. RCM is characterized by, restrictive left ventricle filling and major diastolic dysfunction leading to heart failure and potential transplant at a young age. All three have an autosomal-dominant inheritance pattern with ~50 % of offspring affected with variable penetrance and expressivity, leading to heterogeneous disease presentation and progression, even within the same family [16, 19–23].
Multiple explanations can account for the heterogeneous presentation including gene dosage, location of the mutation, age-related protein quality control, posttranslational modifications, modifier genes and other environmental factors [4]. Adding to the complexity of these diseases is that multiple mutations have been identified in some individuals, leading to increased severity of disease in some cases [24]. With the increase in genetic testing of family cohorts, more patients are identified with a causative sarcomeric mutation while not exhibiting the phenotypic morphological changes that clinically define these diseases. These patients are referred to as genotype positive-phenotype negative [4, 16, 25, 26]. Although they do not present with the normal diagnosis phenotype (i.e., morphological features), they present with subtle, altered systolic function (DCM) or impaired relaxation and abnormal ECGs (HCM) [3, 4, 26–28]. This implicates the intrinsic functional alteration on the sarcomere by the mutant protein as a determinant of whether a dilated or hypertrophic phenotype presents. As such, a deeper understanding of the mutation’s structure/function relationship in the context of the sarcomere is needed to know how to treat these patients prior to presentation of the morphological disease phenotype.
Sarcomere Mutations
DCM
Sarcomere mutations make up an estimated 40 % of genetic DCM with other cytoskeletal, sarcolemmal, metabolic, and nuclear envelope proteins making up the rest [22, 29]. Dominant mutations in actin (ACTC1), myosin-binding protein C (MyBPC3), β-myosin heavy chain (MYH7), α-myosin heavy chain (MYH6), troponin I (TNNI3), troponin C (TNNC1), troponin T (TNNT2), α-tropomyosin (TPM1), and titin (TTN), all can lead to DCM (◘ Fig. 10.3) [29]. Titin truncations make up ~25 % of genetic DCM while the remaining sarcomeric mutations are single missense mutations or small insertions/deletions [22, 29]. Titin functions as a molecular ruler, regulating sarcomere length, and it provides elasticity and passive force to the sarcomere [30, 31]. Little direct research has been conducted on the TTN truncating mutations which cause DCM, but they are hypothesized to alter sarcomere assembly, interactions, and signaling. As such, this chapter’s analysis of molecular mechanisms of sarcomeric DCM will not include these truncations.


Fig. 10.3
Cardiomyopathy mutations : Multiple genetic loci converge on the sarcomere. Schematic representation of the sarcomere with boxes highlighting the thin filament and thick filament proteins. Listed are the sarcomeric proteins that have been implicated as disease causing in the three sarcomeric cardiomyopathies: DCM, HCM, and RCM. Actin, beige; Tm, blue; TnT, yellow; TnC, light red; TnI, cyan; Titin, maroon; MHC, red; MyBP-C, green. Adapted from source [88]: Davis J, Westfall MV, Townsend D, Blankinship M, Herron TJ, Guerrero-Serna G, Wang W, Devaney E, Metzger JM. Designing heart performance by gene transfer. Physiol Rev. 2008;88(4):1567–651
Many but not all sarcomeric mutations that cause DCM result in decreased Ca2+ sensitivity of the myofilament. Thin filament reconstitution assays showed that multiple DCM mutations decrease the Ca2+ affinity of cTnC with increased Ca2+ dissociation rates and decreased Ca2+ association rates [32, 33]. Assays using the entire myofilament showed multiple DCM mutations alter the Ca2+ sensitivity of force and ATPase activity [32, 34, 35]. Although these assays do not recapitulate all aspects of cardiac myocyte function, they do reveal the most basic biophysical role of the mutations, with the only variable being the mutation itself.
Moving to more complex systems, cTnC mutations were transduced into adult cardiac myocytes . Both permeabilized and membrane-intact cellular function revealed decreased Ca2+ sensitivity of force development, decreased contractile amplitude, and faster relaxation [36]. These cellular phenotypes all indicate altered sarcomere function with an inability to activate contractility within the normal physiologic range. Knock-in and transgenic mouse models of cTnT, myosin, and Tm DCM mutations revealed similar phenotypes to human patients with left ventricular dilation, systolic dysfunction, and decreased Ca2+ sensitivity of force development in isolated myofibers [27, 37–40]. These studies have built a model of sarcomeric DCM as a Ca2+ desensitized myofilament, leading to decreased contractility, decreased systolic function, and pathologic remodeling.
HCM
With a prevalence of 1 in 500 people, hypertrophic cardiomyopathy is the most common inherited cardiomyopathy. Clinically, it is defined by asymmetric left ventricular hypertrophy and diastolic dysfunction, with a high propensity for arrhythmias and sudden cardiac death. HCM is the leading cause of sudden cardiac death in the young and in athletes [4, 19]. The first gene mutation implicated in this disease was found in MYH7 [1], [2]. Since that time, more than 1400 mutations in 11 genes have been identified as causative for HCM [16]. An estimated 60 % of HCM is caused by sarcomeric gene mutations, with MHY7 and MyBP-C3 accounting for up to 80 % of those, while TNNT2, TNNI3, and TPM1 make up about 10 % [41]. Excluding MyBP-C3 mutations, which often result in truncated proteins and haploinsufficiency, the vast majority of mutations are missense or small insertion/deletions [3]. The mutant proteins act as poison peptides through incorporation into the sarcomere to dominantly alter sarcomere function.
Early in vitro motility assays, in combination with Ca2+ sensitivity of force development, identified an increase in Ca2+ sensitivity and force development as the primary common biophysical phenotype for most HCM mutations [27, 42, 43]. Ca2+ affinity assays showed increased Ca2+ affinity with decreased dissociation rates for many HCM mutations, suggesting that altered Ca2+ binding by cTnC is the end effector of sarcomere function [33]. Studies using membrane-intact adult cardiac myocytes transduced with mutant TNNI3 or TPM1 genes showed increased contractility, slower relaxation, and altered Ca2+ cycling [36, 44, 45]. Further work with knock-in or transgenic mouse models with MYH7/MYH6, MYBPC3, TNNC1, or TNNT2 mutations revealed in vivo hypertrophy, diastolic dysfunction, Ca2+ cycling abnormalities, arrhythmias, and metabolic inefficiency [42, 46–55]. All of these phenotypes recapitulate the human disease, making these preclinical models appropriate to study the molecular mechanisms of disease.
From a structural perspective, each mutation affects the sarcomere in a unique way, making the location of the mutation an important factor in determining the impact on sarcomere function. Most mutations alter protein-protein interactions that are crucial for activating/inactivating contractility. For example, the majority of mutations in TNNI3 are all located in critical regions of the protein that interact with actin, cTnC, or Tm [8]. In the end, the common denominator of these mutations is to alter cTnC’s affinity for Ca2+, which in turn determines the time of cross-bridge cycling and the rate of relaxation. This is the basis for increased force generation and diastolic dysfunction seen in animal models and human mutation carriers.
The increased Ca2+ affinity of the sarcomere also results in secondary effects, such as increased arrhythmias and decreased energy efficiency, which are common phenotypes in HCM patients [11, 49, 56]. Through the use of a cTnT mutant transgenic mouse and small-molecule Ca2+ sensitizers, it was determined that Ca2+ sensitization leads to increased arrhythmogenesis [57]. Further research determined that the Ca2+ buffering by the sarcomere and focal energy deprivation lead to increased arrhythmogenesis in independent but parallel mechanisms [58, 59]. Through the use of a small-molecule Ca2+ sensitizer in normal wild-type hearts, these studies directly link Ca2+ sensitization to decreased energy efficiency and increased arrhythmias. In addition, with the use of a small-molecule Ca2+ desensitizer in an HCM model, the increase in arrhythmic potential could be abrogated [59]. These studies highlight how Ca2+ sensitivity can lead to many of the common clinical phenotypes associated with HCM patients.
RCM
Restrictive cardiomyopathy is a rare but devastating inherited disease of the sarcomere. RCM presents with restrictive LV filling and major diastolic dysfunction, leading to heart failure and transplant or death at a young age. To date, RCM-linked mutations have been found in TNNI3, MYH7, TNNT2, and ACTC [60, 61]. Several reports provide evidence for overlap between HCM and RCM, both clinically and genetically. At least two separate mutations in TNNI3 have been associated with HCM and RCM. D190G was associated with a mix of HCM and RCM in a 12-member family [62]. R145W was associated with RCM and HCM in two separate families, and R145G and R145Q have been linked to HCM, making codon 145 a hotspot for HCM and RCM mutations [62, 63]. These clinical findings suggest that, in addition to the location of the mutation, the amino acid that replaces the endogenous amino acid is critical to the end phenotype and that environmental, epigenetic, and modifier genes can alter the phenotypic presentation.
As with the other sarcomeric cardiomyopathies, RCM mutations alter the Ca2+ sensitivity of the sarcomere. Similarly to HCM, RCM mutations lead to increased Ca2+ sensitivity but to a much larger extent. In vitro experiments using myofilaments with reconstituted mutant proteins showed increased Ca2+ sensitivity of ATPase and force development [64]. Further research showed increased Ca2+ affinity of cTnC with a cTnI mutation, suggesting a similar molecular signature with HCM [65]. In addition, many of the TNNI3 mutations alter the ability of cTnI to fully inhibit myosin binding to actin, resulting in increased diastolic tone and slower relaxation [64]. Several studies compared HCM and RCM mutations. In vitro Ca2+ sensitivity of force and ATPase assays differentiated the RCM-linked mutation R145W cTnI compared to the HCM-linked mutation R145G [66, 67]. The RCM mutation had a greater increase in Ca2+ sensitivity of force and ATPase and increased maximal force development compared to the HCM mutation at the same codon. Acute genetic engineering of adult cardiac myocytes was used to compare three HCM mutations to three RCM mutations in cTnI [45]. Membrane-intact sarcomere dynamics and Ca2+ transient analysis showed normal contractile amplitude, slow relaxation times, and increased Ca2+ decay rates for all mutations, with the RCM mutations altering these parameters to a greater extent. Further work showed that most RCM mutations and some HCM mutations alter the resting sarcomere length, indicating increased diastolic tone [45, 68, 69]. Transgenic mice expressing R193H cTnI show higher end-diastolic pressures and a progressive diastolic dysfunction with age [69, 70]. These in vivo phenotypes could be explained by the increased Ca2+-independent diastolic tone that could result in restrictive filling of the LV.
Genotype-Phenotype
Although morphological features have historically categorized sarcomeric cardiomyopathies into DCM, HCM, and RCM, the molecular boundaries separating these phenotypes are complex and difficult to compartmentalize. As indicated above, these three morphologically distinct phenotypes share similar genes and a similar molecular end effector (sarcomere Ca2+ affinity). All three are predominately inherited as an autosomal-dominant mutation that results in a poison peptide capable of altering sarcomere function through incorporation into the sarcomere. Heterogeneous presentation of disease can be explained, in part, through the location of the mutation, which can result in differing levels of Ca2+ sensitization/desensitization as discussed above. With a similar molecular mechanism based on multiple preclinical models from reconstituted thin filaments up to genetically engineered rabbit myocytes, the current clinical definition of these diseases needs to be examined. Preclinical evidence points to morphological features of sarcomeric cardiomyopathies as a late-stage phenotype, with abnormalities in Ca2+ sensitivity occurring much earlier.
With advances in genetic testing, more family members of patients with sarcomeric cardiomyopathies are being identified as carriers before any morphological phenotype is present [16]. As the genotype positive-phenotype negative population expands, new clinical markers are needed to track disease progression and early treatment efficacy before morphological changes manifest. These markers should be informed by the basic science that has defined multiple intrinsic properties of these mutations in terms of physiologic measures. Although myofilament Ca2+ sensitivity cannot be measured in a noninvasive way, alternate markers of diastolic and systolic function, in addition to noninvasive P31 magnetic resonance spectroscopy (MRS) to monitor energetic alterations, may enhance our ability to monitor early-stage functional changes in patients.
Experimental Therapeutics
Current therapies in the clinical setting primarily treat symptoms of sarcomeric cardiomyopathies and do not alter the fundamental genetic basis of disease. Increasing identification of genotype-positive individuals without morphological evidence of disease poses both opportunities and challenges for physicians caring for these patients. The potential to treat patients early and prevent or delay the onset of clinical symptoms is a major advantage of genetic testing. A number of early therapeutic targets have been identified, including changes in myofilament Ca2+ sensitivity, metabolism, vascular function, and ion channel function [3]. However, many sarcomeric cardiomyopathy mutations can result in phenotypically different outcomes, with different ages of onset and different severities. In some cases, carriers never develop clinical disease [42]. Modifier genes, epigenetic changes, and environmental factors can all lead to a heterogeneous clinical presentation even among members of the same family [71].
These factors, in addition to the lack of early disease markers, make evaluating treatment efficacy before the onset of fulminant disease difficult. Making treatment decisions before the presence of a clinical phenotype is very challenging for patients and families. Yet a growing body of research seeks to target subclinical manifestations of sarcomeric cardiomyopathies. As knowledge of genotype-phenotype transition and early disease markers increases, early treatment of sarcomeric cardiomyopathies will become an increasingly viable option for patients [4].
Normalizing Ca2+ sensitivity has been investigated as one early intervention that may potentially alter the course of sarcomeric cardiomyopathy development. In a proof-of-principal experiment, dual gene transfer of a Ca2+ sensitized cTnI mutant with a Ca2+ desensitized cTnC mutant in adult cardiac myocytes resulted in functional effects that were normalized toward controls compared to either mutant alone [36]. This suggests that normalizing Ca2+ hypersensitivity with a desensitizer may have therapeutic benefit. Drug screens have led to identification of a number of potential compounds, most of which are still in the preclinical stages of research. These include molecules that directly modulate the function of myosin or the troponin complex, or the phosphorylation of these proteins [72]. One of these compounds, the cardiac myosin activator omecamtiv mecarbil, has been evaluated in clinical trials for systolic heart failure [73]. Recently, it was tested in a preclinical model of DCM with a tropomyosin E54K mutation and resulted in increased myofilament Ca2+ sensitivity [74]. Another Ca2+ sensitizer, propyl gallate [75], was found to decrease remodeling and increase survival in a DCM mouse model with knock-in of cTnC-∆K210 [37], suggesting an important mechanistic role of myofilament Ca2+ sensitivity in sarcomeric cardiomyopathies’ disease pathology. One challenge to overcome with Ca2+ sensitizer and desensitizer therapy as a treatment for preclinical disease is overcompensation. Today, titration of these treatments is difficult due to the lack of clinical markers to assess myocardial Ca2+ sensitivity .
Targeting ion channels is another therapeutic strategy for sarcomeric cardiomyopathies. Increased intracellular Ca2+ can result from myofilament Ca2+ sensitization, which can lead to CAMKII upregulation and activation of phosphorylated ion channels, manifesting as electrophysiological abnormalities [3]. The Ca2+ channel blocker diltiazem prevented hypertrophy, fibrosis, and hemodynamic abnormalities in a preclinical model of HCM [76]. Diltiazem has also been tested in a pilot clinical study to determine whether it can delay the onset of a phenotype in genotype-positive, phenotype-negative patients. Patients were of ages 5–39 and treated for 1–3 years. The drug was well tolerated, and there were minor improvements in left ventricular end-diastolic dimension. Data stratification revealed that patients with MYBPC3 mutations responded more favorably to treatment than patients with MYH7 mutations [77]. This study is most notable for its long-term treatment aiming to prevent a disease phenotype that was not present at baseline.
Vascular abnormalities and metabolic inefficiency are often present early in sarcomeric cardiomyopathies and, therefore, may also be therapeutic targets that could change disease outcome [3]. Perhexiline, originally used as an anti-anginal agent, has been tested in a clinical trial of HCM patients. Perhexiline changes myocardial substrate utilization, decreasing lipid oxidation and increasing glucose oxidation, which results in decreased oxygen consumption [78]. In HCM patients, perhexiline normalized diastolic filling during exercise and increased the PCr/ATP ratio and peak VO2 [79]. Future studies should test long-term outcomes of perhexiline treatment in sarcomeric cardiomyopathies and whether it can delay the onset of hypertrophy in subclinical disease.
An increasing amount of preclinical research on sarcomeric cardiomyopathies focuses on gene therapeutic strategies. Gene therapy has the advantage of correcting the primary cause of the disease, rather than treating functional abnormalities resulting from the mutated gene. Gene therapeutic strategies thus far have focused on increasing the amount of the non-mutated protein contained in the sarcomere. This has been accomplished by either decreasing expression of the mutant allele or increasing expression of the non-mutated protein. The overarching goal of gene therapy in the context of sarcomeric cardiomyopathies is to change the natural history of the disease, reducing its severity or preventing the onset of the disease phenotype [3].
To date, messenger ribonucleic acid (mRNA) trans-splicing [80], exon skipping [81], and gene replacement [53] have all been studied as potential therapies for knock-in mice with a mutant form of MYBPC3. The G > A transition in MYBPC3 exon 6 in this model leads to the presence of multiple disease-causing mRNA variants and decreased total cMyBP-C protein expression and incorporation into the sarcomere. All three strategies served to increase expression of wild-type cMyBP-C transcript or a naturally occurring functional variant. However, only delivery of full-length WT-cMyBP-C resulted in sufficient protein expression and sarcomeric incorporation to improve functional outcomes in mice up to 34 weeks of age [53]. In this study, cMyBP-C was delivered via AAV9 to 1-day-old neonatal mice. The treatment dose dependently increased mRNA and protein expression and decreased expression of disease-causing variants. This coincided with a significant decrease in left ventricular hypertrophy and some improvement in echocardiographic and hemodynamic measures [53]. Increasing expression of the non-mutated protein, as was done in these studies, is ideal for haploinsufficient mutations or in cases where the diseased allele is not preferentially incorporated into the sarcomere, allowing the wild-type protein to outcompete the mutated protein for limited positions within the sarcomere .
In another mouse model of sarcomeric cardiomyopathy expressing the R403Q mutation of myosin heavy chain (MHC), a gene-silencing approach was attempted [82]. RNA interference (RNAi) was delivered via AAV9 to silence the mutant allele. Although transcript expression of MHC-R403Q was decreased by less than one-third with RNAi treatment, this was sufficient to prevent hypertrophy, fibrosis, and prolongation of the QRS interval. RNAi in this context did not reverse hypertrophy nor was it able to prevent its development as the mice aged to 11 months [82]. One limitation of treatment with RNAi against a diseased allele is the need to develop and optimize an RNAi sequence for each patient. On the other hand, this strategy offers promise for those with haploinsufficient sarcomeric cardiomyopathy mutations and a high penetrance of the diseased allele.
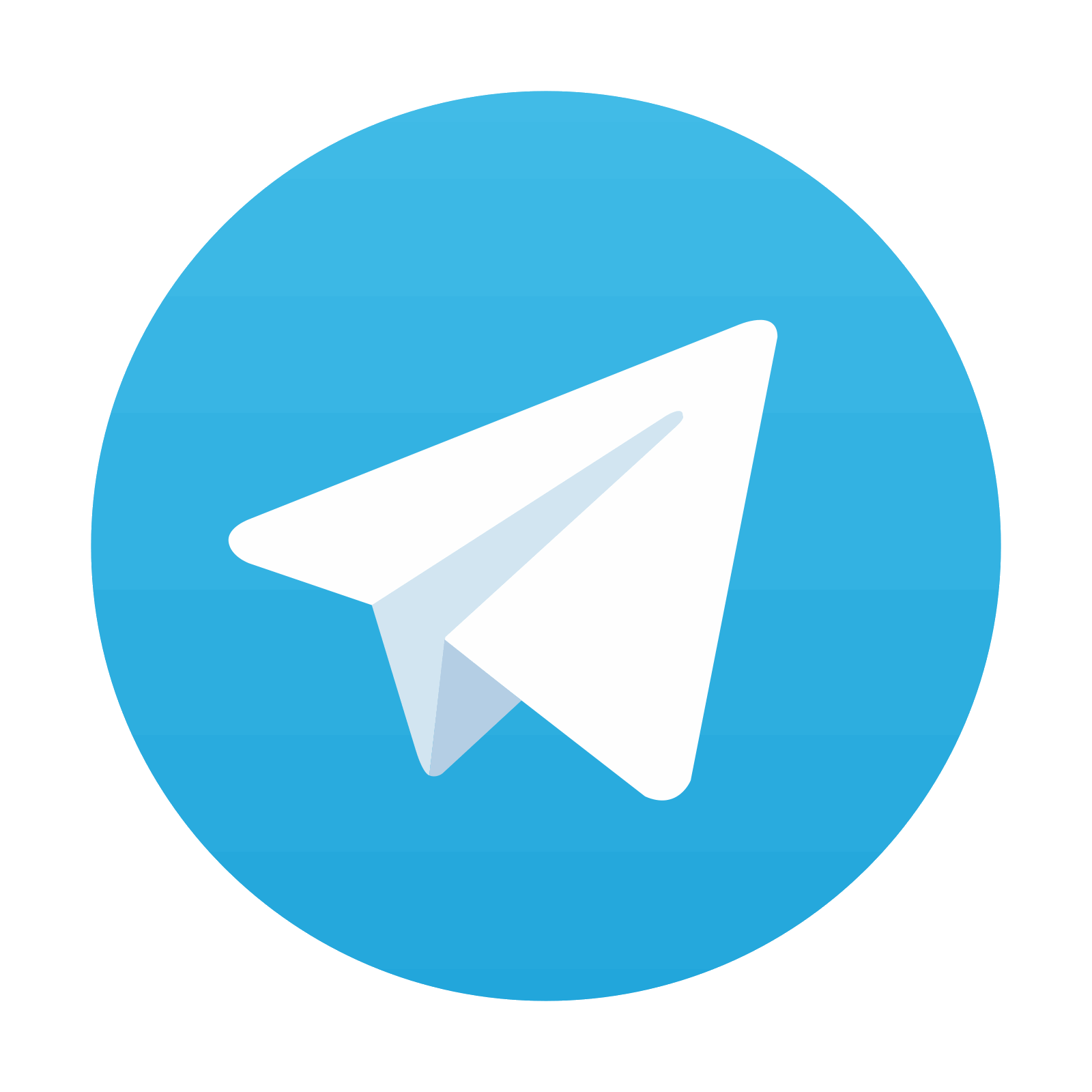
Stay updated, free articles. Join our Telegram channel
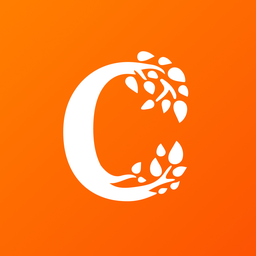
Full access? Get Clinical Tree
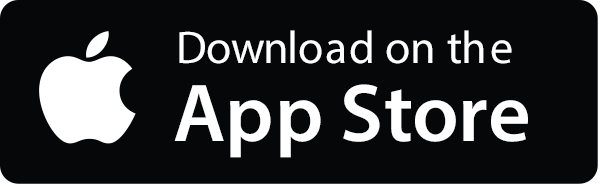
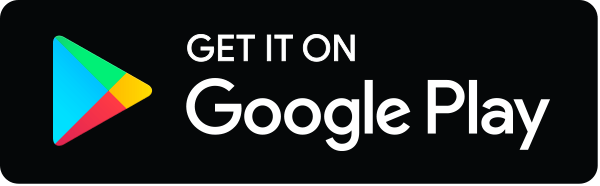