Fig. 1.1
Physiologic and pathologic hypertrophic signaling
Mechanical stretch also activates G-protein-coupled plasma membrane receptors such as AT1R and endothelin A (ETA) in cardiomyocytes and vascular smooth muscle cells, leading to indirect activation of TRP channel opening [24, 41]. Stretching of ventricular cardiomyocytes or treatment with AT-II activates AT1R, inducing activation of extracellular signal-related kinases (ERKs) and Gαq isoforms. Pressure overload in an animal model causes AT1R–dependent cardiac hypertrophy, again even in the absence of AT-II [24]. Gαq proteins activate phospholipase C, leading to an increase in cytosolic inositol triphosphate (IP3) and DAG [42]. IP3 activates TRP 3, 6, and 7, and also triggers an IP3 receptor on the endoplasmic reticulum (ER), leading to Ca2+ release from the ER [43]. This Ca2+ store depletion activates plasma membrane store-operated Ca2+ channels (SOCs), increasing Ca2+ influx from extracellular sources [44] (Fig. 1.2).


Fig. 1.2
Cardiac mechanotransduction
Increased cytosolic concentrations of Ca2+ and DAG activate protein kinase C (PKC) isoforms whose signaling increases expression of early stress-response genes, such as c-fos, c-jun, jun-B, c-myc, egr-1, and heat shock protein (HSP) 70 [45]. Nkx2.5 and GATA-4, also activated by PKC signaling, may participate in PKC down-regulating expression of the sarcoplasmic reticulum (SR) Ca2+ ATPase (SERCA), which is responsible for rapid reuptake of Ca2+ into the SR [46, 47]. SERCA downregulation explains much of the calcium signaling abnormalities seen in heart failure and has emerged as a potential therapeutic target [48, 49]. Changes in calcium handling associated with cardiac hypertrophy are directly involved in the cell death that characterizes the transition to systolic dysfunction and clinical heart failure [50] (Fig. 1.3).


Fig. 1.3
Pathways of cellular death in heart failure
In experimental models and in limited human data, treatment with the calcineurin inhibitors cyclosporine A or FK506 opposes the hypertrophic phenotype [51–54]. Increased cytosolic and mitochondrial Ca2+ concentrations provoke apoptosis [55, 56]. Calcineurin may link hypertrophy to apoptosis by dephosphorylating the pro-apoptotic protein Bcl-2-antagonist of cell death (BAD), promoting its association with Bcl-xl [57].
The actions of GATA-4 and NFAT are opposed by glycogen synthase kinase 3β (GSK3β), which inactivates these proteins through phosphorylation [58]. GSK3β is itself controlled by Akt and PKA, both of which repress GSK3β activity through phosphorylation [59, 60]. Active GSK3β is known to be a negative regulator of hypertrophy [61].
Mechanical stress upon the intact heart activates MAP kinases p38 and ERK 1/2, resulting in phosphorylation of the zinc-finger transcription factor GATA-4. As a result of phosphorylation at serine 105, GATA-4 is activated, thereby allowing its binding to DNA targets such as the BNP promoter [62–64].
AT1R is induced by pressure overload, and the resulting increase in signaling is prohypertrophic in ways that are dependent and independent of AT-II [24]. AT1R signaling activates TRPC channels, resulting in prohypertrophic signals and derangements in calcium handling [43]. AT-II induces expression of TGF-β1 in cardiac fibroblasts, leading to increased collagen production and fibrosis [65, 66].
At a gross level, cardiac hypertrophy strongly predicts the development of clinical heart failure [67]. The pathologic hypertrophy of pressure or volume overload differs molecularly in important ways from the physiologic hypertrophy that occurs in response to exercise training or pregnancy. Re-expression of the fetal gene program appears to be absent in physiologic hypertrophy [68]. Both physiologic hypertrophy and normal cardiac growth are mediated by insulin-like growth factor 1 (IGF-1), which induces hypertrophy through an Akt pathway. Unlike pathologic hypertrophy mediators, IGF-1 activity is anti-apoptotic and IGF-1 administration in a rat model of ischemic cardiac failure leads to a preservation of systolic function and has beneficial effects on adverse cardiac remodeling [69]. Sustained experimental activation of IGF-1 pathways can, however, eventually cause a transition from physiologic hypertrophy to a more classically pathologic phenotype (Table 1.1).
Table 1.1
Cellular and molecular changes in physiologic hypertrophy and heart failure
Normal | Physiologic stimuli | Heart failure | |
---|---|---|---|
Molecular changes | |||
“Fetal gene program” | – | – | Increased |
Myosin heavy chain isoform | α-MHC | α-MHC | β-MHC |
Biomarkers | |||
BNP | – | Unclear | Elevated |
Troponin | Normal | Unknown | May be increased |
Cytokine activation | Normal | Normal | Increased TNF-α, IL-6 |
Catecholamine levels | Normal | Normal | Increased |
Cellular changes | |||
Cell size | Normal | Hypertrophied | Hypertrophied |
Fibrosis | Normal | Normal | Increased |
Cell death | Normal | Normal | Increased |
Capillary density | Normal | Preserved | Decreased |
Calcium cycling | Normal | Normal | Increased |
Metabolism | Predominately fatty acid oxidation | Predominately fatty acid oxidation | Predominately glucose oxidation |
Contractile function | Normal | Normal | Decreased |
Excitation-contraction coupling | Normal | Normal | Altered |
While most cardiac mass is represented by the cardiomyocytes, several other cell types and extracellular structures are important in the control of cardiac function and demonstrate significant alterations in heart failure. Cardiac mast cells, fibroblasts, conductive tissue, endothelial cell, vascular smooth cells, cardiac progenitor, and resident macrophages contribute cardiac mass and biology. Fibroblasts outnumber cardiomyocytes roughly threefold in the intact heart, multiply in response to mechanical stretch, and contribute to extracellular remodeling.
Diastolic stretch activates NADPH oxidase 2 (NOX2), leading to the production of reactive oxygen species (ROS). ROS may then increase Ca2+ release from sarcoplasmic reticulum by sensitizing ryanodine receptor type 2 channels (RyR2) [70]. NOX2 activity may be critical to induction of cardiomyocyte apoptosis by hypertrophic stimuli such as AngII, as NOX2 inhibition or deletion of the NOX2 cofactor p47phox interferes with AngII-induced apoptosis [71].
Myocyte hypertrophy is generally characterized by the reactivation of a number of genes that are normally expressed during fetal development but eventually become quiescent after birth [72]. This “fetal gene program” includes increased expression of GATA-4, Nkx2.5, and PTX transcription factors, is typical of cell cycle entry. These transcription factors drive the expression of classic fetal genes that are markers and essential mediators of cardiomyocyte hypertrophy, including atrial natriuretic (ANF), brain-type natriuretic peptide (BNP), α-skeletal actin, β-myosin heavy chain (βMHC), and angiotensin-II receptor type 1 (AT1R) [73, 74].
Myocardium-Vascular Mismatch
It has been observed that as cardiac hypertrophy progresses, there is relatively lower concomitant vascularization, such that the number of capillaries per area of myocardium declines [75]. Microvascular dysfunction and decreased coronary flow reserve are observed in experimental and clinic hypertrophy and heart failure even in the absence of coronary atherosclerosis [76, 77]. These changes have been linked to subendocardial ischemia in hypertrophy and may accelerate the transition to heart failure. Treatment of a rabbit TAC model with vascular endothelial growth factor (VEGF) induces MMP-2-dependent angiogenesis, delays the onset of LV dilation, and preserves LV contractility [78].
Fibroblast Proliferation and Fibrosis
The majority of cardiac cellular components are represented by cardiomyocytes, endothelial cells, vascular smooth muscle cells, and fibroblasts. But while cardiomyocytes are the necessary cells for cardiac contraction, they comprise only a third of cardiac cells. The remainder of cardiac mass is mostly comprised of fibroblasts, which are responsible for the production of the extracellular matrix (ECM) that functions as the cardiac mechanical scaffold. Additionally, ECM components contribute to the regulation of cellular growth, tissue differentiation, and angiogenesis. Cardiac fibrosis, which is characterized by the increased extracellular presence of collagens and other ECM components, is a hallmark of advanced hypertrophy and heart failure [79].
Fibroblasts can be activated by increased interstitial fluid flow to produce collagen type III and TGF-β, effects that may be opposed by blockade of the AT1 and TGF-β receptors [80]. Experimental hypertrophic stimuli such as infusion of AngII increase fibroblast production of IL-6, which leads to collagen I production from fibroblasts and may contribute to cardiomyocyte hypertrophy through paracrine signaling [81–84].
Excitation-Contraction Coupling
Cardiac contraction results from Ca2+−dependent interactions of myosin and actin myofilaments. During cell membrane depolarization, Ca2+ enters the cardiomyocyte through L-type Ca2+ channels (LTCCs) located at transverse tubules, which are in proximity to the sarcoplasmic reticulum (SR) [85]. Ca2+ influx triggers a larger release of Ca2+ from the SR through ryanodine receptors (RyR2). The SR Ca2+−ATPase (SERCA2A) is responsible for restoring Ca2+ to the SR [48]. The disappearance of SERCA2A during the progression of hypertrophy and heart failure alters the coupling of Ca2+ flux to the cardiomyocyte contractile apparatus [86]. In failing human hearts, there is a significant reduction in t-tubule-SR junctional dyads and thus in the co-localization of LTCCs and RyR2, indicating a degree of both physical and biochemical uncoupling of excitation from contraction [87, 88].
Sarcolemmal Proteins
Sufficient myocyte contractile force is dependent upon appropriate excitation-contraction coupling, which requires precise coordination between the entry of extracellular Ca2+ into the cytoplasm and the wave of Ca2+ release from the sarcoplasmic reticulum (SR) [89]. Transverse tubules (t-tubules) are invaginations of the surface membrane, continuous with the extracellular space, that extend into the interior of cardiac myocytes [36, 90]. T-tubules form a highly organized network that is physically coupled to the SR at Z-discs [91]. This organization is essential for coordination of excitation-contraction coupling. LTCCs are predominately localized to t-tubules and are responsible for the initial cellular Ca2+ influx. Ca2+ efflux is largely handled by the sodium-calcium exchanger (NCX), found on both tubular and non-tubular sarcolemmal [92]. The relative importance of Ca2+ efflux through these different domains is unclear. A population of β adrenergic receptors is localized to t-tubules, where they appear to modulate LTCC function and localize cAMP signaling [93]. In rodent heart failure models, this localization is lost, with β2 receptors redistributing from t-tubules to the cell crest, leading to diffusion of cAMP signaling [94].
Hypertrophied and failing hearts demonstrate misshapen and dilated t-tubules and tubular disorganization and disappearance, which has been called t-tubule remodeling [87]. The degree of tubular remodeling is associated with the severity of heart failure [95]. As L-type calcium channels are primarily localized to T-tubules, there is also a decline in inward calcium currents in failing myocytes [96]. In a rodent model, T-tubule disruption can be reversed after mechanical unloading with heterotopic abdominal heart transplantation [97].
Myocardial Metabolism in Heart Failure
Myocardial metabolism depends to large parts (>70 % at baseline) on the utilization of fatty acids for oxidative metabolism and ATP generation. The complete oxidation of one molecule of palmitic acid as an example for the most prevalent circulating and nutritionally derived saturated fatty acid generates 129 ATP molecules. In contrast, complete oxidation of glucose results in generation of 36 ATP molecules. Therefore, oxidation of fatty acids is a more energy efficient process compared to the oxidation of glucose (Fig. 1.4).


Fig. 1.4
Cardiac metabolism
The reversion to the fetal gene expression in cardiac hypertrophy and heart failure encompasses not only structural and contractile genes but also metabolic substrate utilization. During fetal development, cardiac metabolism depends primarily upon glycolysis, but the heart transitions toward fatty acid oxidation in the postnatal period [98]. While lipid metabolism may increase early in experimental hypertrophy, expression levels of fatty acid oxidation enzymes are decreased in the failing heart [99, 100]. An important determinant of fatty acid utilization and metabolism is the activity of PPARα, which targets binding sites in the promoters for the genes encoding carnitine palmitoyl transferase-І (CPT-І) and long-chain and medium-chain acyl-COA dehydrogenases (LCAD and MCAD) and activates their transcription [101]. In the hypertrophied heart, the relative shift from fatty acid to glucose utilization occurs in concert with decreased PPARα activity and expression [102]. Of note, further changes in myocardial gene expression result in a preferential generation of ATP through anaerobic metabolism, namely glycolytic breakdown of glucose to pyruvate and lactase. This is best known for myocardial metabolism in circumstances of ischemia and has been shown to produce only 8 molecules of ATP during glycolysis resulting in a severe energy (ATP)-depleted state of the failing myocardium. Reversibility of cardiac metabolism after cardiac recompensation requires up to 5 days until ATP stores reach levels known from stable heart failure patients.
Part of the repression of PPARα activity in cardiac hypertrophy may be explained by sequestration of this nuclear transcription factor in the cytoplasm by the MAP kinase kinase MEK1 [103]. The repression of PPARα expression and activity may be involved in the progression of pathological hypertrophy. Administration of PPARα ligands such as conjugated linoleic acid opposes cardiac hypertrophy in spontaneously hypertensive rat strain [104].
Ectopic accumulation of lipids in non-adipose tissues leads to lipotoxicity. In animal models and in human heart failure patients, fatty acid utilization declines and cardiac metabolism shifts back toward the fetal phenotype of glucose dependence [99, 105]. Recent work has demonstrated the myocardial accumulation of toxic lipid intermediates such as ceramide and diacylglycerol in the failing myocardium with depletion of stores of neutral lipids including triglycerides and fatty acids. Mechanical support with a left ventricular assist device (LVAD) may reverse some of the cardiac and systemic metabolic abnormalities observed in heart failure [106].
Myocardial Inflammation in Heart Failure
The progression of cardiac hypertrophy and heart failure are linked to inflammation in several ways. Elevated serum levels of TNF-α and IL-6 are seen among heart failure patients and correlate with NYHA heart failure class, the degree of cardiac cachexia, and overall prognosis [107–110]. Myocardial tissue from patients with severe heart failure obtained at the time of heart transplantation revealed an increased presence of macrophages and T lymphocytes and more endothelial activation, as indicated by the presence of intercellular adhesion molecule-1 (ICAM-1) [111].
Experimental pressure overload induces cardiac expression of proinflammatory cytokines TNF-α and IL-6, which further contribute to cardiac hypertrophy and heart failure [112–114]. TNF-α binding to its receptor TNFR1 leads to formation of the death inducing signaling complex (DISC), downstream caspase activation, and resulting apoptotic cell death. Upregulation of IL-6 expression through an α1 adrenergic receptor-A kinase anchoring protein (AKAP)-inhibitor of IκB kinase β (IKKβ) may lead to autocrine and paracrine increases in fetal and hypertrophic gene transcription through the IL-6 receptor [115]. Patients who respond clinically to cardiac resynchronization therapy show decreased serum levels of IL-6, as well as IL-8, TNF-α, and TGF-β; among non-responders, TGF-β levels increase [116, 117].
Cell Death
The processes outlined above contribute to apoptosis, necrosis, and autophagy, which together account for a significant increase in myocardial cell death and partly account for the transition from compensated hypertrophy to decompensated heart failure. All of the types of cell death can occur simultaneously and in close proximity to each other in failing human hearts [118]. Autophagy is the packaging of intracellular components into double-membrane autophagosomes that are then degraded by lysosomes. Autophagy can be induced by nutrient depletion, hypoxia, oxidative stress, organelle damage, and protein aggregation through pathways that are dependent upon and independent of molecular target of rapamycin (mTOR). Under conditions of ischemia, autophagy appears to be instrumental in maintaining cardiac function. With reperfusion, the continued activities of autophagic processes may lead to cell loss and resulting cardiac dysfunction. One of the pathways responsible for autophagy is AMPK. A mouse model with dominant-negative AMPK in cardiac myocytes showed decreased autophagy in response to ischemia, leading to worse cardiac function in response to myocardial infarction [119]. Some lines of evidence suggest that autophagy facilitates the development of heart failure. Mice with cardiac overexpression of beclin 1, required for autophagosome formation, have increased cardiac autophagy, ventricular dilation, cardiac fibrosis, and mortality compared to non-transgenic mice in response to transaortic constriction (TAC). Mice heterozygous for a disrupted beclin 1 gene have less cardiac autophagy and better preservation of systolic function [120]. Autophagy is repressed by mTOR, a member of the PI3K-related kinase family. Mice with a conditional cardiac knockout of raptor, an essential component of mTOR complex 1, develop severe cardiac dilation, increased autophagy, and a transition from oxidation of fatty acids to glucose utilization in response to TAC; these mice do not develop a classic initial hypertrophic response [121] (Fig. 1.3).
Increased cardiomyocyte apoptosis has been confirmed in multiple animal models of heart failure and in myocardial samples from heart failure patients [122, 123]. Important contributors to cardiomyocyte apoptosis include cytokines that are produced as part of the initial hypertrophic gene expression cascade. Serum TNF-α levels are increased among heart failure patients and are associated with worsening functional class [108, 124–126]. TNF-α expression is increased in mechanically stretched cardiomyocytes in culture and in pressure-overloaded cardiac tissue after aortic banding, correlating with apoptotic index [127, 128]. The apoptotic response is attenuated in TNF-α-knockout mice [127]. Infusion of animal models with TNF-α or cardiac-specific overexpression of TNF-α increases myocyte apoptosis and causes worsening of systolic function [129]. TNF-α binds to its receptor TNFR1, prompting formation of the death inducing signaling complex (DISC), recruitment of FADD, and activation of caspase 8, which then cleaves caspase 3, leading to activation caspase activated DNAse (CAD) and resulting DNA cleavage and apoptotic cell death [130, 131].
Cardiomyocyte necrosis due to oncotic cell death results from cellular ATP depletion, causing inactivation of ATP-dependent ion pumps and thus dissipation of energy-dependent osmotic gradients [132, 133]. Cellular swelling results and intracellular contents are released into the extracellular space. Oxygen deprivation may be a cause of ATP depletion. Decreased oxygen tension can result from coronary ischemia. Additionally, there may chronic hypoxia due to mismatch between myocyte size and vascular density in the hypertrophied heart. In advanced cardiac hypertrophy, the increased size of myocytes outpaces the production of new capillaries, leading to a relative decrease in capillary density and creating increased oxygen diffusion distance to myocytes [75]. There is also an increase in coronary vascular resistance [134].
Cellular necrosis has traditionally been considered to be an accidental and unregulated process but, in at least some cases, appears to be governed by sophisticated regulatory pathways. A plasma membrane pathway, involving some of the same molecular machinery utilized by TNF-α to promote apoptosis, has been identified [135]. The actions of TNF-α at the plasma membrane can promote either cellular survival or death, with necrosis prevailing when survival and apoptotic pathways are inhibited [136]. TNF-α binds to TNF-α receptor I (TNFRI), leading to the recruitment of a multimolecular complex that ultimately activates NF-κB, promoting cellular survival [137]. This complex can subsequently recruit Fas-associated protein with death domain (FADD), resulting in a second complex that can promote necrosis [138, 139].
The coordinated effects of metabolic derangements, cardiomyocyte death, and interstitial fibrosis cause a shift from compensated hypertrophy to decompensated heart failure. PPARγ coactivator-1 (PGC-1) isoforms α and β are both suppressed in response to experimental pressure overload, and the loss of these activities appears central to the hypertrophy-heart failure transition [140, 141]. In addition to regulating the expression of nuclear genes responsible for fatty acid import and oxidation, PGC-1 isoforms also activate the expression of transcription factors that target the mitochondrial genome, controlling the expression of oxidative phosphorylation genes and mitochondrial biogenesis [140, 142]. Cardiac PGC-1α overexpression causes unchecked mitochondrial proliferation, disrupting normal contractile structure and leading to a dilated cardiomyopathy [143]. PGC-1β-knockout mice demonstrate more cardiac fibrosis, higher levels of reactive oxygen species, and greater reduction in ejection fraction after trans-aortic banding than do wild-type mice [141].
Extracellular Matrix Changes in Heart Failure
Extracellular matrix turnover, largely catalyzed by matrix metalloproteinases (MMPs), is central to myocardial remodeling. Myocardial levels of collagenase-3 and membrane-type MMPs are increased in heart failure. Circulating levels of MMP-2 and MMP-9 are found in heart failure patients. Peroxynitrite, formed by the reaction of superoxide anion with nitric oxide, activates MMPs. Conversely, MMP inhibition lessens to effects of peroxynitrite on contractile dysfunction in isolated cardiomyocytes [144]. Animal models demonstrate the ability of pharmacologic MMP inhibition to favorably affect post-MI remodeling. Genetic deletion of tissue inhibitors of metalloproteinases (TIMPs) causes acceleration of post-MI remodeling.
Vascular Changes and Endothelial Dysfunction
Normal endothelium functions as a structural barrier between blood and the vascular wall and as a regulator of vascular tone and coagulation by balancing opposing factors [145]. One of its most important roles is the production of nitric oxide (NO) by nitric oxide synthase (NOS) isoforms. NO produces vascular smooth muscle relaxation through stimulation of guanylate cyclase production of cyclic guanosine monophosphate (cGMP). The oxidative stress that characterizes heart failure inhibits NOS production of NO. Decreased NO availability then contributes to vasoconstriction and increased after load. Vasoconstriction as well may cause a relative hypoperfusion of the hypertrophied myocardium, further contributing to ventricular dysfunction.
Impaired Nitric Oxide Coupling
In heart failure, there appears to be increased NOS production of reactive oxygen species (ROS) such as superoxide anion instead of NO, a development that has been termed NOS uncoupling. The simultaneous decline in NO and increase in ROS lead to a decline in vasodilation and myocardial relaxation [146]. The reaction of ROS with NO leads to the formation of peroxynitrite. Infusion of peroxynitrite into intact rat hearts causes production of pro-MMP-2, a transient vasodilation that is then followed by a sustained vasoconstriction, and progressive mechanical cardiac dysfunction, changes that are prevented by treatment with an MMP inhibitor and by quenching peroxynitrite with glutathione [147].
Biomarkers of Myocardial Remodelling
As proposed by Braunwald, biomarkers of heart failure may be classified into those that reflect myocyte injury, myocardial stretch, oxidative stress, neurohormonal activation, ECM remodeling, inflammation, renal dysfunction, and others that don’t fit neatly into these categories [148].
B-type natriuretic peptide (BNP), most recently more commonly used in clinical practice, and atrial natriuretic peptide (ANP) are the major examples of biomarkers reflecting myocardial stretch. The value of natriuretic peptides in guiding heart failure management has been established by clinical studies [149]. Further, plasma levels of the soluble form of ST2, which is a myocardial receptor for IL-33 in its membrane-bound form, are increased in response to myocardial stretch, and predict the occurrence of heart failure among patients with an ST-segment elevation myocardial infarction (STEMI), and death among patients with established heart failure [150, 151].
Circulating cardiac troponins (Tn) I and T are the major clinical markers of myocardial injury and necrosis. Elevated serum troponin levels can be found among individuals with essential hypertension and cardiac hypertrophy [152–155]. Conventional serum TnI levels correlate with LV wall thickness among patients with hypertrophic cardiomyopathy (HCM) [156]. In a separate study, increasing high-sensitivity TnT (hs-TnT) levels correlated with worsening functional status, outflow obstruction, and with left ventricular wall thickness among HCM patients [157]. Serum TnI predicted the development of heart failure among a community-based sample of elderly men, independently of its association with blood pressure, body mass index, smoking, and history of myocardial infarction [158]. High-sensitivity TnT level associates with risk of death among patients with nonischemic dilated cardiomyopathy [159].
The serum ratio of pro-collagen type I amino-terminal propeptide (PINP) to collagen type I cross-linked carboxyterminal telopeptide (CITP), respective markers of collagen synthesis and breakdown, may predict collagen accumulation [160]. ECM turnover depends upon the activity of matrix metalloproteinase (MMP) isoforms, which belong to a large family of zinc-dependent proteases. Their activities are inhibited by tissue inhibitors of metalloproteinases. A study of patients with heart failure with preserved ejection fraction showed significant differences in levels of MMP-2, TIMP-4, and pro-collagen type III amino-terminal propeptide (PIIINP) compared to patients with LVH but not heart failure and with controls; in levels of MMP-3 and MMP-8 compared to patients with LVH alone; and in levels of MMP-7, MMP-9, TIMP-1, TIMP-2, CITP, and osteopontin. A model consisting of PIIINP, MMP-2, MMP-8, and TIMP-4 and adjusted for clinical covariates demonstrated an improved ability to distinguish patients with heart failure with preserved ejection fraction compared to a model consisting of clinical covariates alone [161].
Serum C-reactive protein (CRP) levels are elevated among heart failure patients compared to people without heart failure. Furthermore, higher CRP levels are associated with more severe heart failure and with a higher risk of death [162]. Higher levels of CRP and IL-6 predict the onset of CHF among patients with and without metabolic syndrome [163–165]. In one study of patients with severe heart failure, serum levels of TNF-α and IL-6 and soluble receptors differed significantly between survivors and nonsurvivors, higher levels predicting lower likelihood of survival [166]. Serum levels of TNF-α and of its soluble receptors (types I and II) were elevated among heart failure compared to age-matched healthy controls, and levels of the type-II soluble receptor (sTNFRII) independently predicted death [110]. Among patients with severe heart failure in a separate study, levels of IL-6, TNF-α, and IL-1β and of the soluble receptors for TNF-α and IL-2 were associated with risk of death in univariate analyses; IL-6 levels independently predicted a combined endpoint of death, new heart failure episodes, and need for heart transplantation [167]. Galectins are a family of β-galactoside-binding proteins that regulate inflammation [168]. Higher galectin-3 levels are associated with worse heart failure functional classification and with worse outcome [169, 170].
Neuregulin-1 (NRG1) is produced by coronary endothelial cells and bind to ErbB4 receptors on cardiomyocytes, prompting its interaction with HER2/neu (ErbB2) and triggering of downstream effectors such as focal adhesion kinase (FAK) that are involved in sarcomere organization and cell survival [171]. Antagonism of HER2 with the monoclonal antibody trastuzumab in addition to chemotherapy increased the risk of cardiac fourfold compared to chemotherapy alone, despite an overall oncologic and survival benefit [172]. NRG-1β levels increase with heart failure class and predict transplant-free survival [173]. In a small clinical trial, daily infusion with a recombinant human NRG-1 for 11 days produced beneficial acute hemodynamic effects (significant reductions in pulmonary capillary wedge pressure, systemic vascular resistance, and serum levels of norepinephrine and aldosterone; and increased cardiac output) and a sustained 12 % increase in LV ejection fraction at 3 months [174].
Cardiac Non-coding RNA
Micro-RNAs (miRs) are noncoding molecules, 18 to 25 nucleotides in length, that silence expression of specific genes by binding to complementary segments of messenger RNA, targeting them for degradation and/or inhibiting their translation [175]. Studies comparing human heart failure and animal models have found concordance in the upregulation of mir-24, -195, 199a, and 214 in response to cardiac stress [176].
MiRs have been found to circulate stably in blood [177]. MiR-423-5p is increased in the failing human heart; plasma levels distinguish heart failure patients from healthy controls and patients with dyspnea not due to heart failure and correlated with N-terminal-pro-BNP [178]. A small clinical study demonstrated that a combination of serum levels of miR-423-5p, -320a, -22, and -92b could differentiate between heart failure patients and controls, although the interpretation is limited by significant differences between the groups in drug treatment and the prevalences of diabetes and chronic kidney disease [179]. In a separate study, miR-423-5p showed no significant differences among patients with transposition of the great arteries and a failing systemic right ventricle after atrial repair and sex- and age-matched health controls [180]. The logarithm of plasma miR-126 concentration negatively with log[BNP], declined with worsening NYHA class, and increased with clinical improvement [181]. Plasma levels of miR-499 were elevated after acute MI and viral myocarditis and during acute heart failure [182].
Conclusion
Much progress has been made in the past half-century in the understanding of the molecular changes preceding and accompanying heart failure. Continued investigation is hoped to identify new targets for prevention, diagnosis, and therapy. Studies of in vitro systems have been invaluable in unraveling the molecular and cellular mechanisms at play in heart failure. But the major breakthroughs in this area and in biomedical science generally have issued from the creation of genetically manipulated animal models. The availability of human genome sequences and our deepening understanding of inter-individual genetic variability will illuminate new discoveries in heart failure risk and treatment. Epigenetic elements, such as microRNAs, especially those that circulate in plasma may prove to be both markers and mediators of heart failure risk, progression, and response to treatment. The explosion of data resulting from all of these efforts will require advances in bioinformatics and in systems biology.
References
1.
He J, Ogden LG, Bazzano LA, Vupputuri S, Loria C, Whelton PK. Risk factors for congestive heart failure in US men and women: NHANES I epidemiologic follow-up study. Arch Intern Med. 2001;161:996–1002.PubMed
2.
Rich MW. Epidemiology, pathophysiology, and etiology of congestive heart failure in older adults. J Am Geriatr Soc. 1997;45:968–74.PubMed
3.
Kannel WB, Ho K, Thom T. Changing epidemiological features of cardiac failure. Br Heart J. 1994;72:S3–9.PubMedPubMedCentral
5.
Ruwhof C, van der Laarse A. Mechanical stress-induced cardiac hypertrophy: mechanisms and signal transduction pathways. Cardiovasc Res. 2000;47:23–37.PubMed
6.
Tallarida RJ, Rusy BF, Loughnane MH. Left ventricular wall acceleration and the law of Laplace. Cardiovasc Res. 1970;4:217–23.PubMed
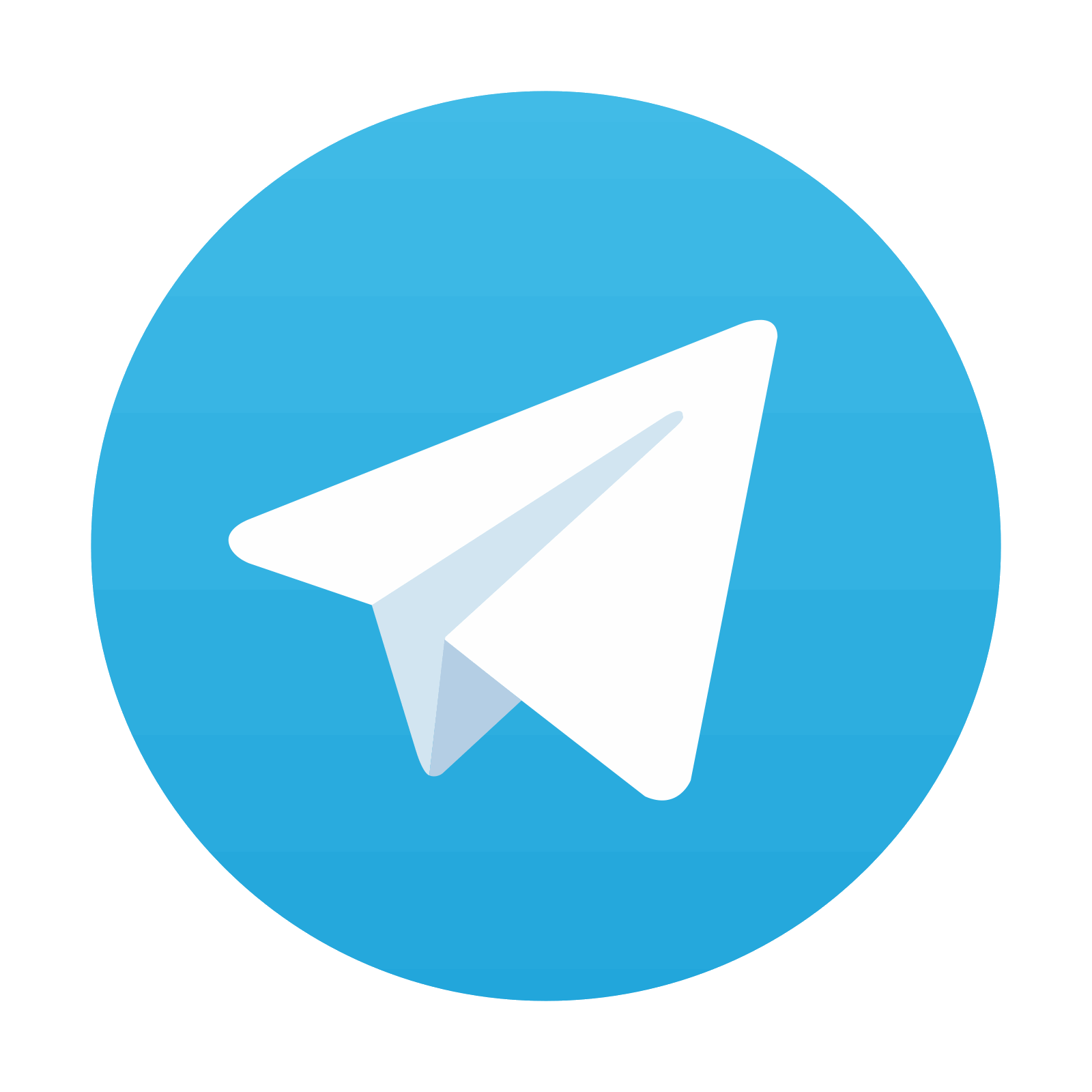
Stay updated, free articles. Join our Telegram channel
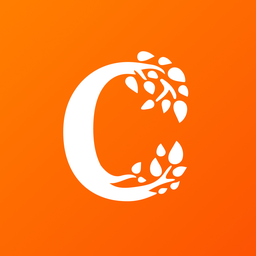
Full access? Get Clinical Tree
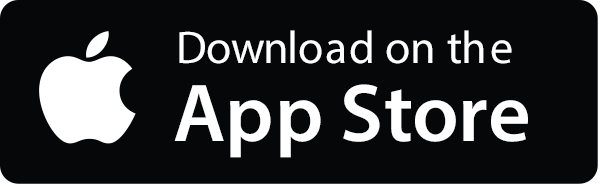
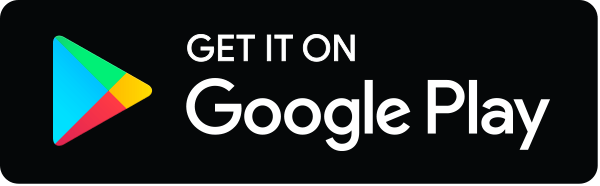