Fig. 3.1
The molecular structure of DNA from nucleotide to chromosome. DNA at the molecular level is composed of nucleotides, which consist of a nitrogenous base (adenosine, cytosine, guanine, and thymine), a deoxyribose sugar, and a phosphate group. The nucleotides form complementary base pairs via hydrogen bonding. The base pairs form the double-stranded helical structure of DNA with a sugar-phosphate backbone. Segments of DNA form genes that encode for proteins and have coding segments called exons and noncoding segments called introns. The DNA is wrapped around histone proteins and further packaged into chromatin. The DNA containing chromatin is then further packaged into chromosomes
This double-helix structure of DNA is important in storing and replicating biological information, given that the DNA backbone is resistant to cleavage and the double strands of DNA each store the same biological data. In total, 3.2 billion bases of DNA provide a blueprint for the synthesis of all proteins in the human body.
Promoters, Enhancers, and Repressors
The DNA structure enables it to serve as a template for storing biological data. Transcription is the first step in gene expression, where ribonucleic acid (RNA) is synthesized via RNA polymerase using the DNA template (◘ Fig. 3.2). For initiation of transcription, an RNA polymerase (RNAP II) binds to the double-stranded DNA in the promoter region. Promoter sequences are regions of DNA that define where the initiation of transcription by RNA polymerase begins. Promoter sequences are located at the 5′ end of the transcription initiation site, and they define the direction of transcription and which strand of DNA is transcribed.


Fig. 3.2
Representation of the central dogma of biology. DNA is located within the nucleus of the cell and transcribed into pre-messenger RNA. The pre-messenger RNA is further processed into mature messenger RNA and, subsequently, is exported out of the nucleus into the cytosol. The messenger RNA is then translated into protein via the ribosome
RNA polymerase and transcription factors form a transcription complex and bind to the promoter sequence. The complex unwinds the DNA and allows the RNA polymerase to transcribe a single strand of DNA to RNA [3–5]. Similar to DNA, RNA is composed of nucleotide bases, but RNA polymerase substitutes uracil (U) in RNA for thymine (T) moieties found in DNA. The RNA strand is transcribed in a 3′–5′ direction until it reaches a sequence in the DNA known as the termination sequence that enables the RNA polymerase to detach from the DNA.
The regulation of transcription is critically important as it temporally controls the initiation of transcription and the amount of RNA generated. Transcription of a gene can be regulated by transcription factors that can enhance or repress the gene expression. Enhancers are sites in the DNA helix where activators bind to enhance the interaction between RNA polymerase and a promoter. Enhancers increase the attraction of RNA polymerase for the promoter and/or change the structure of DNA to increase the expression of a gene. Repressors bind to the operator region, a sequence of DNA close to or overlapping the promoter region. The repressor acts to impede the RNA polymerase binding or the progress of transcription, which results in decreased or silenced gene expression. Enhancing or repressing gene expression is crucial to the maintenance of health; variations and abnormalities contribute to individual differences in gene expression and disease, respectively.
Introns and Exons
The initial transcription of DNA to RNA produces an unedited RNA molecule known as pre-RNA. Within the pre-RNA are sequences that code for proteins known as exons and noncoding sequences known as introns . Mature messenger RNA (mRNA) is produced from pre-RNA by splicing, which is the process of removing the noncoding intron sequences. The spliceosome is a complex of proteins and small RNA molecules which excise introns and join together adjacent exon sequences. While introns were previously thought to be useless (or “junk”) sequences, ongoing research has revealed that introns harbor noncoding RNAs, which may have an important role in gene expression and allow for alternative splicing.
Together, the spliced exons represent protein-coding messenger RNA. There are untranslated regions (UTRs)—sequences of mRNA that precede the start codon for protein translation and sequences that follow the stop codon that are not translated into protein. These regions are known as the five prime UTR (5′ UTR) and three prime UTR (3′ UTR) sequences, respectively.
UTR sequences are present in mature mRNA and promote mRNA stability. The mature mRNA also has a 5′ cap composed of a specifically altered guanine nucleotide and, at the 3′ end, a chain of adenine nucleotides known as the poly(A) tail. These additional modifications to the mature mRNA enable attachment of the ribosome for protein translation, transport of mRNA, and prevention of mRNA degradation. Subsequently, the mature mRNA is transported out of the nucleus and is translated to protein by ribosomes. The mRNA has protein information encoded by RNA sequences that are read by ribosomes in a grouping of nucleotide triplets termed codons. Each codon represents a specific amino acid and the specific sequence of amino acids is determined by the mRNA sequence.
MicroRNA-Mediated Gene Regulation
While molecular biology primarily focused on protein-encoding genes, recent discoveries of microRNAs (miRNAs) and noncoding RNAs have indicated that these sequences play important roles in the regulation of gene expression [6]. MicroRNAs are a recently identified class of short, noncoding RNAs that impact the regulation of gene expression at the posttranscriptional level. MicroRNAs are embedded within the introns of the pre-mRNA and frequently have similar expression profiles as the mRNA. MicroRNA genes are transcribed by RNA polymerase II as a large pri-microRNA with a hairpin loop (◘ Fig. 3.3).


Fig. 3.3
MicroRNA biogenesis . The production of mature microRNA begins with production of the primary (pri)-microRNA transcript via RNA polymerase. The pri-microRNA is further processed to a short hairpin structure called pre-microRNA by the Drosha nuclease. The pre-microRNA is exported from the nucleus to the cytoplasm. In the cytoplasm, the pre-microRNA complexes with the RNase dicer, which cleaves the microRNA to its 21–23 nucleotide mature length. The functional single strand of mature microRNA interacts with the RNA-induced silencing complex (RISC) to guide mRNA cleavage, translational repression, and gene silencing
The pri-microRNA is further processed by an RNase III enzyme known as Drosha, which creates a smaller ~70 nucleotide precursor-microRNA (pre-microRNA). The pre-microRNA is transported to the cytoplasm where it interacts with dicer, another RNase II enzyme, which cleaves the hairpin to form a single-strand, mature microRNA of 21–25 nucleotides. Mature microRNA is incorporated into the RNA-induced silencing complex (RISC). MicroRNAs bind to the 3′ UTR of the target mRNA and form heteroduplexes with the target sequences.
The binding of the microRNA is mediated by the seed sequence, which is a 2- to 8-nucleotide sequence of the miRNA that recognizes the mRNA target and promotes binding [7]. The RISC mediates gene silencing via microRNA base pairing with complementary sequences in mRNA.Therefore, the mRNA can be silenced by either cleavage of mRNA, destabilization of mRNA, or less efficient translation to proteins.
The role of microRNAs in the heart has received intense interest and is hypothesized to play an important role in cardiovascular development or in response to stressful stimuli. Deletion of the microRNA processing Dicer gene in mice resulted in severe heart failure and premature death [8]. Similarly, inhibition of the microRNA miR-1, the most abundant microRNA in the heart, led to perturbed cardiac development [9].
Recent studies have also identified specific microRNAs that mediate changes in transcript expression in cardiovascular disease states. In a seminal study regarding microRNAs and cardiovascular disease, a group of stress-induced microRNAs were identified to be up- or downregulated in response to transverse aortic banding in a mouse model of cardiac hypertrophy [10]. These investigators also over-expressed microRNA miR-195, which was upregulated in hypertrophy, and were able to induce a heart failure state in animal models. MicroRNA profiling has also been conducted in various heart failure states and significant microRNA expression changes have been noted. Ikeda et al. have analyzed microRNAs in human left ventricular samples from patients with ischemic cardiomyopathy, dilated cardiomyopathy, and aortic stenosis [11]. These investigators detected 87 microRNAs that were differentially expressed, including the upregulation of the prohypertrophic miR-214 and the downregulation of the anti-hypertrophic miR-71–76 in dilated cardiomyopathy and aortic stenosis.
In another study of failing human left ventricular myocardium, investigators demonstrated an upregulation of microRNAs that reactivate the fetal gene program [12]. Thum et al. also demonstrated that the majority of microRNAs that are differentially expressed in the failing human heart are similar to those expressed in fetal cardiac tissue, further suggesting a role for microRNAs in the reactivation of the fetal gene program during ventricular remodeling [13]. Further studies focused on microRNA expression, and regulation may enhance our understanding about the identification of microRNA targets and their impact on prognosis and treatment of cardiovascular disease.
Epigenetic Regulation
While gene regulation can be impacted by a number of genetic factors at the transcriptional and translational level, epigenetic regulation involves heritable changes in gene expression without alterations in the DNA sequence that occurs in response to a stimulus. Two major mechanisms of epigenetic regulation involve chromatin remodeling and DNA methylation. Chromatin is a complex of DNA wound around histone proteins. It is the chromatin that forms chromosomes.
First, histones can be posttranslationally modified through methylation and acetylation. The modified histones assume a different configuration and impact the means by which DNA wraps around the histones. Therefore, DNA may be more or less available for transcription, and this epigenetic modification alters the expression of the resultant gene. Second, epigenetic regulation can occur through the methylation of DNA at cytosine bases by DNA methyltransferases. The DNA methyltransferases convert cytosine to 5-methylcytosine. Methylated DNA serves as a docking site for methyl-binding proteins that oligomerize through DNA to recruit chromatin-remodeling complexes. This results in chromatin being less available for transcription, leading to a reduction in gene expression.
Epigenetic inheritance is a mechanism that allows for stable propagation of gene activity states from one generation of cells to the next. It is associated with the differentiation of immature cells in disease states. For example, somatic cells may have an epigenetic mark that restricts expression of genes involved in self-renewal or pluripotency, thus resulting in the terminal differentiation of the cell. These epigenetic mechanisms were apparent in the cloning of the sheep, Dolly (1996) [14]. Here, the nucleus from a differentiated cell was delivered into an enucleated unfertilized oocyte to clone a sheep.
While geneticists were ultimately successful in the cloning of a sheep, the process of somatic cell nuclear transfer (SCNT ; also referred to as cloning) was very inefficient. The research investigators identified that, while the nucleus of the differentiated cell contained the DNA necessary to engineer an entire sheep, it was not easy to revert a fully differentiated nucleus into a pluripotent state. This strategy demonstrated the importance of epigenetic regulation in cell differentiation, which resulted in heritable changes that restricted gene expression patterns and defined cell identify.
While these epigenetic marks are stable, the recent discovery of human-induced pluripotent stem cells establishes the capacity of adult somatic cells (i.e., skin cells from an adult) to revert or reprogram the epigenome of the cell to a more pluripotent state when it is exposed to a cocktail of transcription factors [15–17]. Additionally, Ieda et al. showed that cardiac developmental transcription factors could be used to directly reprogram dermal fibroblasts into differentiated cardiac-like cells, which resulted in an epigenetic shift of cardiac genes [18].
While the epigenome may be able to be reprogrammed to allow for a more pluripotent state, somatic cells may have epigenetic memory, which results in a preference for differentiation to their original cell lineage. Sanchez-Freire et al. derived human-induced pluripotent stem cells from both skin fibroblasts and cardiac progenitor cells and demonstrated that human-induced pluripotent stem cells derived from cardiac progenitor cells differentiated more efficiently into cardiomyocytes [19]. Also, the dermal fibroblast-derived human inducible pluripotent stem cells had increased methylation of the Nkx2–5 gene, which is a critical factor in cardiac development.
Epigenetics may also play an important role in cardiovascular disease development , especially the heart failure state. Movassagh et al. identified three genes having a role in angiogenesis, which were differentially methylated in human heart failure regardless of etiology [20]. Kaneda et al. also identified differential histone modifications associated with human heart failure that impacted significant cardiac signaling pathways [21]. Further understanding of epigenetic regulation in cardiovascular disease may provide important mechanistic insights into environmental stimuli, the development of disease states, and may serve as candidates for novel therapies.
Genomic Approaches to Cardiovascular Disease and Heart Failure
Molecular medicine began with the identification of the structure of DNA in 1953. Nearly 50 years later, in 2001, the sequence analysis of the entire human DNA truly heralded the genomics era. A genomics approach to studying cardiovascular disease requires screening all the genes and gene variants associated with a particular disease state across the human genome. New sequencing technologies have accelerated this approach by making genome sequencing feasible, representing an important advance in further elucidating cardiovascular diseases.
The Human Genome Project began in 1990 as an international collaboration with the monumental goal of decoding the entire human genome, the sum of the genetic material of a human being. The human genome is encoded on 46 chromosomes (23 pairs) consisting of 3.2 billion base pairs of DNA. Molecular biologists and scientists working for the Human Genome Project sequenced the 3.2 billion base pairs in the human genome. Not only did they identify the sequence but also the location of the sequences of DNA on each chromosome and the linkage maps to evaluate genes.
In 2001, Human Genome Project biologists and scientists published a working draft of the human genome [22], and, in 2003, the full human genome was sequenced. The project identified the approximately 20,500 genes in the human genome, which were fewer than the initial prediction of 50,000–100,000 genes. Additionally, the Human Genome Project has spurred identification of specific genes that lead to disease states and pharmacologic therapies specific to genetic mutations. For example, PCSK9 was identified as a gene and a mutation resulted in an autosomal dominant variant of familial hypercholesterolemia [23]. This discovery was made shortly after the human genome was sequenced. Novel pharmacotherapies have been developed to inhibit PCSK9 and have been tested in several clinical trials [24–26].
Not only has the Human Genome Project resulted in identification of disease-causing genes, but emerging technologies have increased the efficiency of genome sequencing. While the initial human genome took more than 10 years to sequence, at a cost of several billion dollars, today, sequencing an individual’s genome can be completed in several days at a cost of less than $8000. Decoding the human genome has proven to be a major advance in genomics, but the real challenge resides in understanding how the sequences of DNA impact human health and disease.
Exome Sequence Analysis
The exome constitutes the protein-coding fraction of the genome—about 1 % of the total human genome. While it represents a relatively small subset of the entire genome, the exome is predicted to harbor up to 85 % of disease-associated mutations [27]. Whole-exome sequence analysis requires the selection of the exome portion of the genome and subsequent high-throughput DNA sequencing. Therefore, whole-exome sequence analysis represents a realistic and cost-effective technique to identify genetic variations that cause genetic and common diseases. Whole-exome sequence analysis has been used to identify novel mutations for patients with familial cardiomyopathy who tested negative on routine genetic testing [28, 29]. Importantly, exome sequence analysis does not include the analysis of introns.
GWAS and SNP Analysis
A genome-wide association study (GWAS ) evaluates a large number of genetic variants to identify associations between specific genetic variants and complex human diseases. The study requires screening a large number of patients affected by a specific disease state, such as non-ischemic cardiomyopathy, and searching for alleles that are more frequently found in affected patients than in healthy individuals. Typically, GWAS focuses on identifying allelic variants known as single nucleotide polymorphisms (SNPs). GWAS relies on data from the Human Genome Project, International HapMap Project, and SNP Consortium, which have identified millions of SNPs [30].
Large genome-wide association studies have identified a significant amount of alleles associated with cardiovascular disease, largely focused on coronary artery disease [31]. Relatively fewer studies have focused on heart failure, but several loci associated with heart failure have been identified. Among these, variations in the BAG3 and HSPB7 genes were found to be associated with dilated cardiomyopathies [32]. Larson et al. performed a GWAS to investigate their association of heart failure in the Framingham Heart Study and identified an SNP, rs939398, which maps to the ryanodine receptor gene and is associated with heart failure [33]. Genome-wide association studies, while useful, have a number of limitations including the need for large sample sizes and the lack of identification of causative genes responsible for disease .
Molecular Signaling in the Normal and Failing Heart
Genetics play a significant role in human cardiovascular disease; however, intracellular signaling pathways play a central role in regulating cardiac function. Adaptations in the signaling pathways in response to stressors can be adaptive, but can also lead to disease states. An enhanced understanding of the normal signaling pathways and the alterations in the pathways that lead to cardiac remodeling and heart failure at the cellular level is critical to elucidating molecular mechanisms and the development of new therapies.
Beta-Adrenergic Signaling
β-adrenergic receptors are members of the G-protein-coupled receptor (GPCR) superfamily and serve as a link between the cardiovascular and sympathetic nervous system. β-adrenergic receptors regulate cardiac contractility, heart rate, and automaticity.
Two major subclasses of β-adrenergic receptors (β1 and β2) exist in the heart, of which the β1-receptor is predominant in the normal, unperturbed adult heart. Stimulation of the β-adrenergic receptors by catecholamines, such as norepinephrine or epinephrine, results in the activation of the stimulatory G-protein (Gs) and adenylate cyclase (AC) signaling pathway, which results in increased cyclic AMP (cAMP) (◘ Fig. 3.4a) [34].


Fig. 3.4
β-adrenergic signaling pathway. (a) β-agonist catecholamines bind to the β1 receptor at the cell membrane. This activates Gαs, which disassociates from Gβ and Gγ, and associates with adenyl cyclase (AC). This leads to the generation of cyclic AMP (cAMP), which in turn activates protein kinase A (PKA). PKA phosphorylates several effector proteins including phospholamban, ryanodine receptor (RYR), voltage-gated L-type calcium channel, and cardiac troponin T (cTNT). These changes lead to increased intracellular calcium, which translates to increased contractility and chronotropy. (b) Chronic β-adrenergic stimulation in heart failure. Chronic β-adrenergic stimulation of cardiomyocytes results in GRK-mediated phosphorylation of the β-adrenergic receptor as well as recruitment of β-arrestin. The inhibitory β-arrestin binding to the β-adrenergic receptor leads to sequestration of the receptor, internalization, and eventual lysosomal degradation. Additionally, chronic β-adrenergic stimulation leads to increased intracellular calcium concentrations, which in turn stimulates the calmodulin-CaMKII pathway. Ultimately, this leads to mitochondrial transition pore opening and eventual cell death
The primary target for cAMP is protein kinase A (PKA). PKA phosphorylates’ key downstream targets include voltage-gated calcium channels, sarco-/endoplasmic reticulum (SR) calcium transport ATPase (SERCA), troponin I, ryanodine receptors, and phospholamban. Phosphorylation of these proteins leads to increased cardiac inotropy and chronotropy through the increase of calcium influx via the voltage-gated calcium channel, increased calcium reuptake into the sarcoplasmic reticulum via SERCA, and the modulation of cardiac sarcomeric calcium sensitivity via troponin (◘ Fig. 3.4a) .
In the failing heart, circulating catecholamines increase in response to increased sympathetic activity. Initially, heightened sympathetic activity is adaptive in boosting cardiac output through increased inotropy and chronotropy. But chronic catecholamine activation can lead to a loss of contractility. In the failing human heart, β1-adrenergic receptors are selectively downregulated and desensitized in an attempt to protect the heart from deleterious signaling due to chronic catecholamine stimulation. Both β1- and β2-adrenergic receptors become uncoupled from the signaling pathways secondary to β1-downregulation, but also, more specifically, due to desensitization via phosphorylation of β-adrenergic receptors by specific kinases, such as GRK family kinases.
A family of inhibitory proteins, β-arrestins, also bind to phosphorylated proteins and prevent activation of G-proteins. This leads to sequestration of β-receptors, internalization, and eventual lysosomal degradation (◘ Fig. 3.4b). The consequence of β-adrenergic downregulation and desensitization is that, for a given level of adrenergic stimulation, less cAMP is produced. This, in turn, leads to decreased activation of PKA, causing decreased downstream effectors.
β-adrenergic receptor blockers are now a standard treatment in heart failure. Multiple trials have shown a significant mortality benefit from β-blockers in heart failure patients (US Carvedilol Heart Failure Study, MERIT-HF, etc.) [52–54]. β-adrenergic blockers can lead to prevention of chronic β-adrenergic stimulation and result in both upregulation of β-adrenergic receptors and resensitization of the receptor.
Calcineurin and CaMK Signaling Pathways
Calcium is a major second messenger in cardiac signaling and has the ability to activate multiple signaling cascades, including the calcineurin and calcium/calmodulin-dependent kinase (CaMK) pathways . Calcineurin, a serine/threonine phosphatase, is activated by binding to calcium-bound calmodulin (◘ Fig. 3.5). It results in dephosphorylation of nuclear factor of activated T cells (NFAT), enabling NFAT to translocate into the nucleus.


Fig. 3.5
Calcineurin and CaMK signaling pathway. Extracellular stimuli that increase intracellular calcium levels result in saturation of calmodulin. Calmodulin binds calcineurin, which leads to activation of calcineurin. Calcineurin dephosphorylates the transcription factor NFAT, which can then translocate into the nucleus. Nuclear NFAT leads to hypertrophic gene expression. Similarly, an increase in intracellular calcium levels also leads to activation of CaMK. CaMKII phosphorylates histone deacetylases, which leads to subsequent derepression of hypertrophic gene expression
Activation of NFAT leads to activation of the myocardial hypertrophy gene pathways via association with a number of transcription factors, including GATA4 and MEF2C. Calcineurin has been implicated in physiological and pathological cardiac hypertrophy and shown to be upregulated in heart failure where it promotes hypertrophy and apoptosis.
CaMK is a family of regulatory enzymes that phosphorylate a number of proteins that modulate cardiac contractility and hypertrophy. CaMKs are autophosphorylated in the presence of increased intracellular calcium. CaMKII is the predominant cardiac isoform, which modulates hypertrophy, contractility, and cell survival via activation of the transcription factor MEF2C results in the activation of the fetal gene program and cardiac hypertrophy. CaMKII is noted to be increased at the transcriptional level, protein level, and during enzymatic activity in the heart failure state [35]. Similar to the impact of calcineurin signaling in heart failure, CaMKII activation can lead to apoptosis and cell death.
MAPK Signaling Pathways
The mitogen-activated protein kinase (MAPK ) cascade consists of a series of successively activated protein kinases (◘ Fig. 3.6). The three major MAPK branches include the extracellular signal regulated kinases (ERKs), c-Jun N-terminal kinases (JNKs), and p38 MAPKs. Mitogenic stimuli and stresses lead to activation of the MAPK signaling cascade via activated G-protein-coupled receptors (GPRCs). The activated Gαq protein activates small G-proteins such as Ras directly via the released Gβγ-subunits, which are activated by growth factors such as EGF, FGF, IGF-1, and TGFβ. The initial MAPK pathway leads to activation of MAPK kinase kinase (MAPKKK), resulting in sequential activation of MAPKK and then effector MAPK kinases (ERK), c-Jun N-terminal kinases (JNKs), and p38. The activated MAPKs are able to phosphorylate transcription factors that have the ability to regulate cardiac gene expression, including hypertrophy and cell survival.


Fig. 3.6
Mitogen-activated protein kinase (MAPK) signaling pathway . The receptor tyrosine kinase (RTK) is activated by the binding of ligands such as fibroblast growth factor, insulin-like growth factor-1, and epidermal growth factor. The activated RTK leads to activation of the GTPase proteins such as Ras and Rho. The activated GTPases stimulate the MAPK signaling cascade. The MAPK cascade is organized into three tiers including MAPKkinase kinase kinase (MAPKKKs) which then activate MAPKinase kinases (MAPKKs) and subsequently activate MAPKinases, which include ERK, p38, and JNK. The activated MAPK can translocate into the nucleus and lead to transcriptional activation of genes including GATA4, C-Jun1, C-myc, C-fos, and CREB
In heart failure, the role of MAPK pathways in the progression of disease is complex due to the intersection and overlap of pathways. However, MAPK pathway activation is known to occur in heart failure, and the p38 and JNK pathways are hypothesized to contribute to pathological remodeling in heart failure [36].
PI3K/Akt Signaling Pathways
Phosphoinositide 3 kinase (PI3K ) and protein kinase B (PKB), which is also known as Akt, are key signaling pathways in cardiac growth and cell survival. PI3K can be activated through various stimuli including insulin, insulin-like growth factor-1, cardiotrophin, β-adrenergic agonists, ischemia, and pressure overload (◘ Fig. 3.7). The activated PI3K forms the second messenger phosphatidylinositol [3,4,5] trisphosphate (PIP3) by binding and phosphorylating PIP2 at the cell membrane.
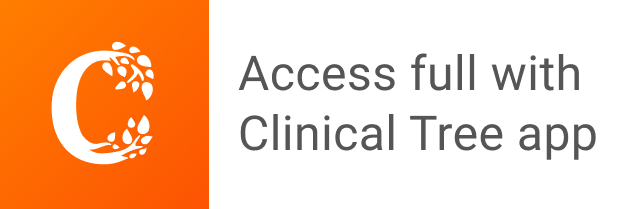