Background
The aortic and mitral valves are anatomically linked through a fibrous continuity. The investigators hypothesized that severe aortic stenosis (AS) would alter this fibrous continuity, affecting both the mitral valve and left ventricular function, and that mitral valve function would be altered after aortic valve replacement (AVR). The aim of this study was to evaluate the impact of AS and its treatment with surgical AVR on the mitral valve.
Methods
Three-dimensional transesophageal echocardiography (using a Philips iE33 system) was performed on 49 patients: 20 controls with normal valves and left ventricular function, 20 with AS and normal left ventricular function studied before and after AVR, and nine with systolic heart failure and normal valves. Custom software tracked the aortic and mitral valves in three-dimensional space, allowing automated measurements of aortic and mitral annular (MA) morphology throughout the cardiac cycle.
Results
Patients with AS before AVR had reduced MA velocities. After AVR, aortic and MA areas were significantly smaller throughout the cardiac cycle compared with controls and pre-AVR values. MA displacement was reduced after AVR and in patients with systolic heart failure compared with those with AS and controls.
Conclusions
Dynamic MA function is changed with AS and after AVR through alterations in the aortic-mitral fibrous continuity. The prosthetic valve ring results in reduced aortic and MA areas, which could affect blood flow in and out of the left ventricle. These changes suggest that the design of future prosthetic aortic valves should be more flexible to preserve the function of the aortic-mitral fibrous continuity.
It is well recognized that the mitral valve (MV) is a complex structure composed of the annulus, leaflets, and chordae and that coordination among these components is required for the valve to function normally. Previous studies describing abnormal MV function have focused on disease states that primarily affect the MV leaflets or apparatus, such as Barlow’s disease and ischemic mitral regurgitation. However, these studies did not take into consideration that the MV is not an isolated structure within the heart. The MV annulus is supported by the left ventricular (LV) myocardium, and the anterior mitral leaflet is in fibrous continuity with the left and noncoronary cusps of the aortic valve. Intuitively, on the basis of the anatomic linkage between the aortic valve and the MV, normal physiologic performance of these two valve would require that they function in a coordinated fashion.
The recently developed real-time matrix-array transesophageal echocardiographic (TEE) transducer allows the dynamic relationship between the aortic valve and the MV to be studied in real time in humans. Using custom software with three-dimensional (3D) TEE imaging, we recently confirmed that normal human MVs and aortic valves function interdependently. Specifically, changes in mitral annular (MA) height, shape, and size during MA contraction may facilitate aortic annular (AoA) expansion and subsequently aortic valve leaflet opening, leading to improved blood flow through the aortic valve during LV ejection. Conversely, AoA contraction facilitates MA expansion, thus improving blood flow through the MV. Knowledge obtained from isolated MV or aortic valve studies suggests that these interdependent changes contribute to the efficiency of valvular and ventricular performance. This valvular coupling has been described in clinical studies reporting decreased MV regurgitation severity after aortic valve replacement (AVR). Overall, the extant knowledge of normal aortic-mitral valvular interaction suggests that disease or surgical processes affecting one valve may have unanticipated adverse effects on the dynamics of the other “uninvolved” valve.
Sclerocalcific aortic stenosis (AS) is the most common valve disease in North America, and treatment involves surgical valve replacement or the more recently introduced transcatheter valve implantation. Although AoA dynamics are known to be altered after AVR, the effects of AVR on MV function are unknown. The aims of this study were to evaluate the impact of sclerocalcific AS in elderly patients on MV dynamics and whether further changes would be observed after AVR. As well, we examined the impact of AVR on systemic arterial compliance.
Methods
Study Design
We studied 49 patients undergoing clinically indicated TEE imaging, who were divided into three groups: (1) controls, (2) patients with sclerocalcific AS studied before and after AVR, and (3) patients with systolic heart failure (SHF) with no AS, included to separate changes in LV function from valvular changes.
Patient Population
Inclusion criteria for the control group ( n = 20) were (1) normal aortic valve, MV, pulmonic valve, and tricuspid valve function and (2) LV ejection fraction (EF) ≥55%. This group was identified from patients undergoing clinically indicated TEE imaging for the assessment of a cardioembolic source of stroke.
Inclusion criteria for the AS group ( n = 20) were (1) AS, defined as an aortic valve area ≤1.0 cm 2 with a mean valve gradient ≥40 mm Hg and/or an aortic jet velocity ≥4.0 m/sec; (2) trileaflet aortic valve; (3) normal MV, tricuspid valve, and pulmonic valve function; (4) LV EF ≥55%; (5) treatment with surgical AVR; and (6) adequate 3D echocardiographic image quality to allow quantification of both valves simultaneously. Patients included in the AS group were required to have LV EFs >55% to ensure that changes in the MV were not due to poor LV systolic function. In total, 32 patients with severe, sclerocalcific AS were screened, and six were excluded for inadequate image quality, five for LV dysfunction, and one for bicuspid valve anatomy.
Inclusion criteria for the SHF group ( n = 9) were (1) LV EF ≤45% and (2) normal aortic valve, MV, pulmonic valve, and tricuspid valve function.
The institutional review board approved this study, and written informed consent was obtained at the time of consent for the clinically indicated TEE studies.
Real-Time 3D Echocardiography
TEE studies were performed using an iE33 ultrasound imaging system, equipped with a matrix-array TEE transducer (Philips Medical Systems, Andover, MA). The probe was positioned at the midesophageal level at a 120° tilt, taking care to include the entire mitral apparatus, aortic valve, and proximal ascending aorta in the pyramidal data set. The mid and apical regions of the left ventricle were excluded to maximize frame rates, which ranged from 17 to 20 frames/sec. A wide-angled, full-volume mode was selected for data acquisition, and electrocardiographic gating was used to merge seven narrow pyramidal scans obtained over seven consecutive heartbeats. For operative candidates, mechanical respiration was suspended during acquisition to reduce stitch artifacts. For controls and patients with SHF, TEE studies were performed under conscious sedation, so multiple 3D data sets were acquired and the data set with stitching external to the region of interest or minimal artifact was selected for analysis.
Image Analysis
Full-volume 3D data sets were digitally stored and transferred to a workstation with custom software designed to quantify the dynamic behavior of the mitral and aortic annuli. This program was developed in collaboration with a biomedical engineering group at the University of Milan and has been used in other studies to examine aortic-mitral coupling. Measurements from this program were validated against manually traced values obtained using QLAB version 8.0 (Philips Medical Systems). Overall, the program functions by semiautomatically detecting the aortic valve and MV and tracking them in 3D space throughout the cardiac cycle. The tracked points were then displayed frame by frame to visually verify their positions, allowing manual correction when necessary.
MV Annular Initialization and Measurement
First, a cut plane representing the LV apical three-chamber view was selected at end-diastole, which was defined as the frame immediately after MV closure ( Figure 1 ). In this plane, two points were identified on the anterior (MA1) and posterior (MA2) mitral annulus ( Figure 1 A). This initialization was then repeated (MA3 and MA4) on a plane orthogonal to the apical three-chamber view ( Figure 1 B) and crossing the middle of the line connecting MA1 and MA2. This middle point was considered the MA center. Subsequently, nine long-axis cut planes evenly rotated around the MA center were automatically displayed. To complete the initialization procedure, the operator selected two points on each plane, one on each side of the mitral annulus ( Figure 1 C). The same procedure was then applied to the isovolumic relaxation frame, which was defined as the frame before MV opening. After automatic tracking, the MA points were connected using spline interpolation to obtain the annular line. Finally, a 3D surface-rendered MA surface was generated for each consecutive frame throughout the cardiac cycle and displayed on the 3D image ( Figure 1 D). The sequence of 3D MA surfaces and annular lines was used to quantify (1) MA surface area, (2) maximal MA longitudinal displacement, (3) maximal anterior MA longitudinal displacement, (4) maximal posterior MA longitudinal displacement, (5) MA intercommissural distance, (6) MA anteroposterior diameter, and (7) MA height. MA height was the maximal vertical distance between the highest (anterior or posterior) and lowest (anterolateral or posteromedial) points of the saddle-shaped MV.

Aortic Valve Annular Initialization and Measurements
In this study, the term “aortic annulus” refers to the virtual line representing the insertion of the aortic cusps into the sinuses of Valsalva. This definition was feasible for bioprosthetic valves, but for mechanical valves, it was defined as the plane of leaflet coaptation. First, two points on the AoA were manually initialized during end-diastole on a cut plane of the volume data set. Then, the orthogonal plane crossing the center of the line connecting these two points was displayed, and two additional points were selected on the aortic annulus. From these four points, the vector orthogonal to the aortic annulus was automatically computed and used as the vertical axis of the aortic annulus. Nine vertical cut planes passing through this axis (20° apart) were displayed one by one, and two AoA points were identified on each plane. Using these additional points, the vertical axis of the aortic annulus was recalculated. The positions of the coaptation point, the three commissural points, and the interatrial septum were manually marked on the end-diastolic frame. The same procedure was then applied to the end-systolic frame, which was defined as the frame immediately preceding MV leaflet opening. These AoA points were automatically tracked throughout the cardiac cycle, and the maximum and minimum AoA projected areas were recorded. Once the aortic and mitral annuli were identified and tracked throughout the cardiac cycle, both 3D surface-rendered annuli were superimposed frame by frame on the 3D images ( Figure 2 ).

AoA-MA Angle
The Mitral-Valve-Quantification package (QLAB) was used to analyze the AoA-MA angle. The AoA-MA angle describes the relationship between the AoA and MA planes. This is best measured using 3D TEE imaging because it relates the individual 3D motion of the aortic and mitral annuli during the cardiac cycle. To obtain this measurement, three orthogonal MA images at end-systole were displayed and subsequently modified to optimize visualization of the entire annulus ( Figures 3 A– 3 C). Four key MA reference points (anterolateral and posteromedial hinge points of the leaflet insertion, anterior and posterior points) as well as the aortic annulus were used for initialization ( Figures 3 A and 3 B). Subsequently, with three key orthogonal planes locked in, 14 markers around the annulus at leaflet insertion were initialized in seven rotational planes ( Figure 3 C). Using these initialization points, the software calculated the AoA-MA angle ( Figure 3 D).

LV Outflow Tract (LVOT) Area
The 3D Quantification package (QLAB) was used to measure the LVOT, located 5 mm below the insertion points of the aortic valve leaflets. Measurement of the LVOT was based on three orthogonal LVOT images, which were displayed and modified to optimize visualization of the entire region ( Figures 4 A–4C). From the plane perpendicular to the LVOT, the LVOT area was manually traced to obtain the area at end-systole and end-diastole ( Figure 4 C). This plane was also used to measure the end-systolic and end-diastolic LVOT long and short axes, which were defined as the widest and the narrowest cross-sectional diameters of the LVOT, respectively.

Systemic Arterial Compliance
Pre-AVR and post-AVR systemic arterial compliance was estimated using the following formula: body surface area–indexed stroke volume divided by pulse pressure. The difference between pre-AVR and post-AVR systemic arterial compliance was then calculated.
Statistical Analysis
The significance of differences between groups of patients and those who received mechanical versus bioprosthetic valves was determined using Student’s t tests. Differences between pre-AVR and post-AVR measurements were tested using paired t tests. Pearson’s correlation was performed to examine the relationship between the difference in pre-AVR and post-AVR systemic arterial compliance and (1) the difference in pre-AVR and post-AVR maximum projected AoA area, (2) the difference in pre-AVR and post-AVR minimum projected AoA, and (3) the difference in pre-AVR maximum minus minimum AoA and post-AVR maximum minus minimum AoA. P values < .05 were considered significant.
Results
Basic demographic characteristics of the patient groups and the results of all measurements are summarized in Table 1 . The mean LV EF was 27 ± 11% in patients with SHF, 60 ± 4% in control subjects, and 64 ± 7% in the AS group. In the AS group, the mean aortic valve gradient and the mean aortic valve area before surgery were 47 ± 11 mm Hg and 0.9 ± 0.2 cm 2 , respectively. The presence of MA calcification and LV hypertrophy was common in patients with AS. Twelve of the 20 patients received Carpentier-Edwards Perimount Magna aortic valves (Edwards Lifesciences, Irvine, CA), two received Medtronic Mosaic Tissue Valve (Medtronic, Inc., Minneapolis, MN), three received MCRI On-X mechanical valve (On-X Life Technologies, Inc., Austin, TX), two received St. Jude Regent mechanical valves (St. Jude Medical, St. Paul, MN), and one received a St. Jude Trifecta bovine valve (St. Jude Medical). For all 20 patients, the average prosthetic valve size was 24 ± 3 mm (range, 21–27 mm). Eleven of the 20 patients underwent coronary artery bypass surgery at the time of AVR. Five of the nine patients with SHF had ischemic cardiomyopathy, one had sarcoidosis, and the remaining three had angiographically proven nonischemic cardiomyopathy. All study patients were in sinus rhythm.
Variable | Controls ( n = 20) | Patients with AS ( n = 20) | Patients with SHF ( n = 9) |
---|---|---|---|
Age (y) | 59.2 ± 17 | 72 ± 9 | 64 ± 10 |
Men | 10 | 14 | 7 |
Body surface area (m 2 ) | 2.1 ± 0.2 | 2.1 ± 0.3 | 2.2 ± 0.2 |
History of coronary artery disease | 0 | 2 | 5 |
Mean aortic valve gradient (mm Hg) | 47 ± 11 | ||
Aortic valve area (cm 2 ) | 0.9 ± 0.2 | ||
LV EF (%) | 60 ± 4 | 64 ± 7 | 27 ± 11 |
More than mild LV hypertrophy | 0 | 8 | 0 |
More than mild MA calcification | 0 | 5 | 0 |
MA Dynamics
Table 2 presents the summary of MA dynamics. In all groups, MA area was largest at early diastole and smallest at midsystole. Throughout the cardiac cycle, MA area was similar among controls and patients with AS before AVR. After AVR, MA area was significantly reduced ( Figure 5 ), with significantly smaller MA intercommissural diameters compared with control and pre-AVR values. Patients with SHF had larger MA areas with wider MA anteroposterior and intercommissural diameters compared with controls and patients with AS before and after AVR.
Measurement | Controls ( n = 20) | Patients with AS ( n = 20) | Post-AVR ( n = 20) | Patients with SHF ( n = 9) |
---|---|---|---|---|
MA area | ||||
Minimum MA area (during systole) (cm 2 ) | 9.8 ± 2.1 | 9.5 ± 1.7 | 8.1 ± 1.7 ∗† | 12.3 ± 1.6 ∗†‡ |
Maximum MA area (during diastole) (cm 2 ) | 11.7 ± 2.4 | 11.2 ± 2.0 | 9.5 ± 1.9 ∗† | 14.2 ± 1.6 ∗†‡ |
Minimum anteroposterior diameter (mm) | 31 ± 4 | 31 ± 4 | 29 ± 5 | 36 ± 5 ∗†‡ |
Maximum anteroposterior diameter (mm) | 36 ± 4 | 35 ± 4 | 33 ± 5 | 41 ± 5 ∗†‡ |
Minimum intercommissural diameter (mm) | 36 ± 5 | 35 ± 4 | 32 ± 4 ∗† | 40 ± 3 †‡ |
Maximum intercommissural diameter (mm) | 41 ± 5 | 40 ± 3 | 36 ± 5 ∗† | 44 ± 4 †‡ |
MA displacement | ||||
Maximum MA displacement (mm) | 9.0 ± 3.8 | 8.8 ± 2.2 | 6.0 ± 1.5 ∗† | 4.3 ± 1.4 ∗†‡ |
Maximum anterior MA displacement | 8.7 ± 3.7 | 8.3 ± 1.7 | 5.4 ± 1.5 ∗† | 5.3 ± 0.9 ∗† |
Maximum posterior MA displacement | 9.7 ± 3.9 | 9.4 ± 2.3 | 6.6 ± 1.9 ∗† | 4.7 ± 1.4 ∗†‡ |
Maximum MA velocity (mm/s) | 1.9 ± 0.9 | 1.2 ± 0.4 ∗ | 1.2 ± 0.3 ∗ | 1.1 ± 0.2 ∗ |
MA height | ||||
Maximum MA height (mm) | 12 ± 3 | 11 ± 2 | 10 ± 2 ∗ | 10 ± 2 ∗ |
† P < .05 versus patients with AS.

MA longitudinal displacement throughout the cardiac cycle was not statistically different between controls and patients with AS before AVR. After AVR, MA displacement was significantly reduced compared with pre-AVR and control patients. This was due to reductions in both the anterior and posterior MA motion. Patients with SHF had smaller MA displacements compared with controls and patients with AS before and after AVR because of reductions in the motion of both the anterior and posterior mitral annulus. Maximal MA velocity was significantly decreased in patients before AVR compared with controls. It remained reduced after AVR and was similar in patients with SHF.
Minimal MA height was similar among all groups. Maximal MA height was similar between controls and patients with AS. After AVR, maximal MA height was significantly reduced. Patients with SHF also demonstrated significantly reduced maximal MA height during the cardiac cycle.
AoA Areas
Reciprocal to the MA area, the projected AoA area was smallest in early diastole and largest at early systole in all groups ( Table 3 ). Throughout the cardiac cycle, AoA area was similar in controls and patients with AS before AVR. After AVR, AoA area was significantly smaller compared with controls, and maximal area was reduced compared with pre-AVR values. Patients with SHF had larger AoA areas compared with controls and patients with AS before and after AVR.
Measurement | Controls ( n = 20) | Patients with AS ( n = 20) | Post-AVR ( n = 20) | Patients with SHF ( n = 9) |
---|---|---|---|---|
AoA area | ||||
Minimum AoA area (during diastole) (cm 2 ) | 4.4 ± 1.2 | 3.7 ± 1.7 | 3.3 ± 1.1 ∗ | 5.3 ± 0.8 ∗†‡ |
Maximum AoA area (during systole) (cm 2 ) | 5.4 ± 1.0 | 5.1 ± 1.1 | 3.7 ± 1.3 ∗† | 6.5 ± 1.0 ∗†‡ |
AoA-MA angle | ||||
Minimum angle (°) | 99 ± 15 | 95 ± 24 | 102 ± 25 | 102 ± 15 |
Maximum angle (°) | 121 ± 17 | 118 ± 27 | 121 ± 31 | 117 ± 21 |
LVOT area | ||||
Minimum LVOT area (during diastole) (cm 2 ) | 3.6 ± 1.0 | 3.4 ± 1.1 | 3.0 ± 1.1 † | 5.3 ± 0.8 ∗†‡ |
Maximum LVOT area (during systole) (cm 2 ) | 4.2 ± 1.0 | 4.1 ± 0.9 | 3.3 ± 1.2 † | 5.5 ± 0.7 ∗†‡ |
Minimum long-axis dimension (cm) | 2.5 ± 0.3 | 2.3 ± 0.5 | 2.2 ± 0.4 † | 3.0 ± 0.4 ∗†‡ |
Maximum long-axis dimension (cm) | 2.5 ± 0.3 | 2.5 ± 0.4 | 2.3 ± 0.4 ∗ | 3.0 ± 0.3 ∗†‡ |
Minimum short-axis dimension (cm) | 1.8 ± 0.3 | 1.8 ± 0.2 | 1.7 ± 0.4 † | 2.2 ± 0.2 ∗†‡ |
Maximum short-axis dimension (cm) | 2.0 ± 0.3 | 2.0 ± 0.2 | 1.8 ± 0.3 ∗† | 2.3 ± 0.2 ∗†‡ |
LV volumes and EF | ||||
BSA-indexed end-diastolic volume (mL/m 2 ) | 64 ± 25 | 77 ± 17 | 60 ± 15 † | 131 ± 23 ∗†§ |
BSA-indexed end-systolic volume (mL/m 2 ) | 25 ± 10 | 31 ± 7 | 25 ± 7 † | 105 ± 24 ∗†§ |
EF (%) | 60 ± 3 | 61 ± 2 | 59 ± 2 † | 24 ± 9 ∗†§ |
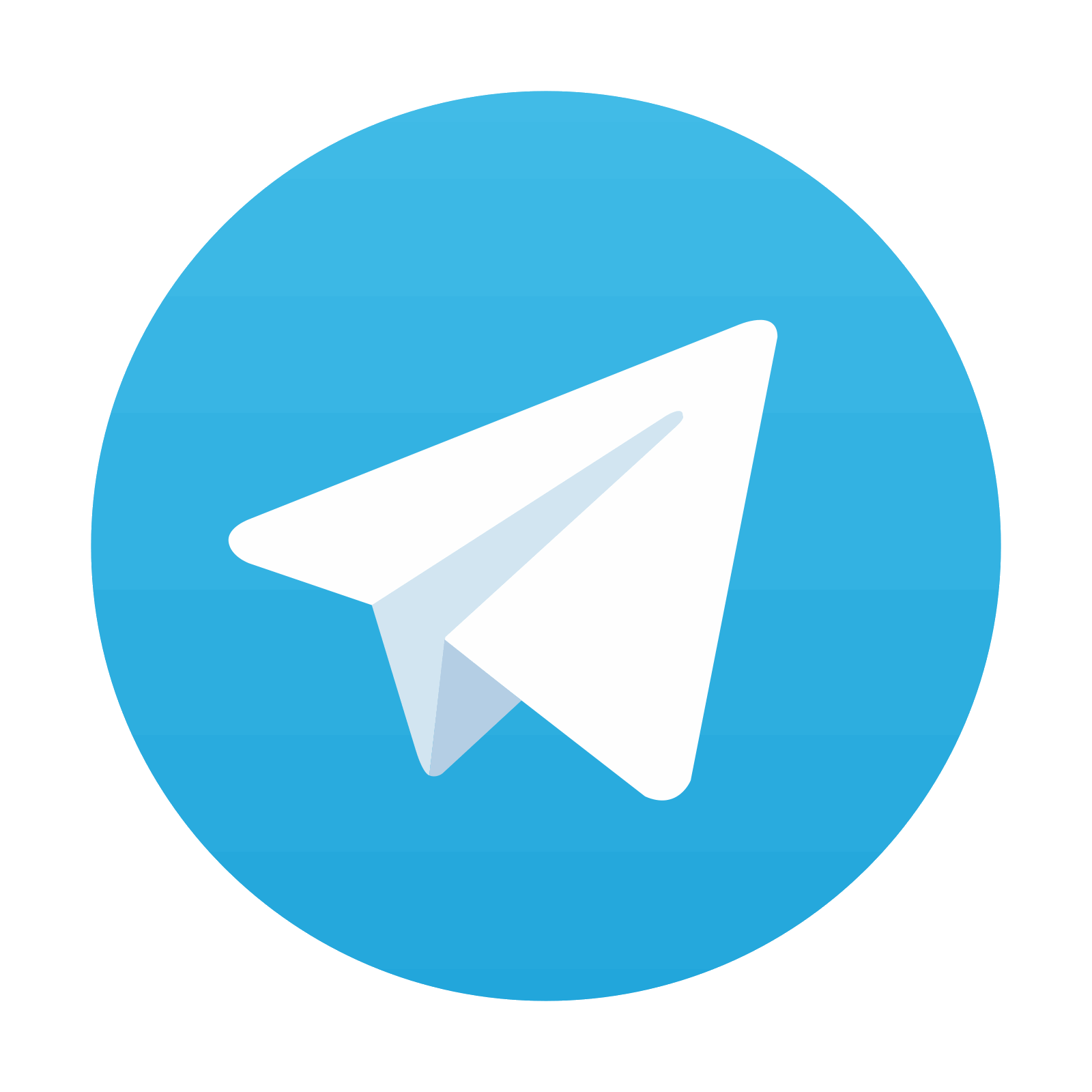
Stay updated, free articles. Join our Telegram channel
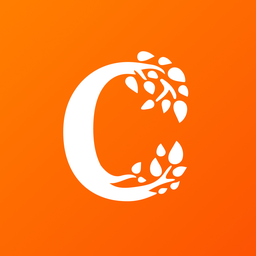
Full access? Get Clinical Tree
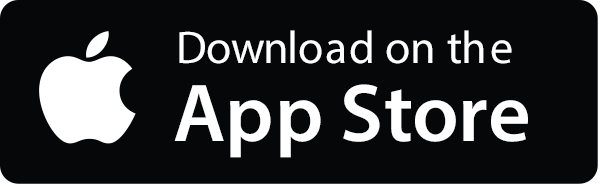
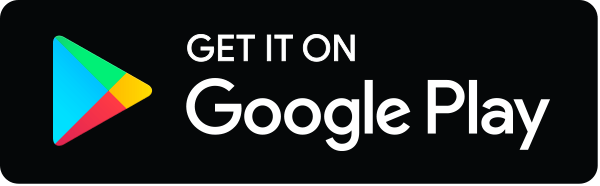
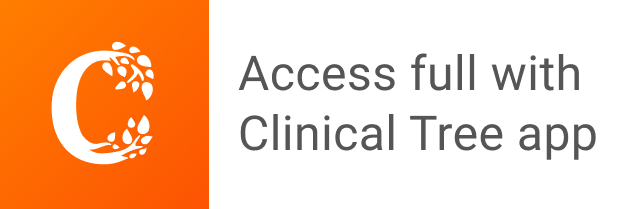