(1)
Project-team INRIA-UPMC-CNRS REO Laboratoire Jacques-Louis Lions, CNRS UMR 7598, Université Pierre et Marie Curie, Place Jussieu 4, Paris Cedex 05, France
Abstract
Mitogen-activated protein kinase (MAPK) modules are commonly used to transduce signals aimed at regulating cell fate (cell differentiation, proliferation, senescence, and death) and inflammation. They mediate responses to extracellular signals, such as hormones, growth factors, and cytokines, as well as stresses and intercellular interactions.
Mitogen-activated protein kinase (MAPK) modules are commonly used to transduce signals aimed at regulating cell fate (cell differentiation, proliferation, senescence, and death) and inflammation. They mediate responses to extracellular signals, such as hormones, growth factors, and cytokines, as well as stresses and intercellular interactions.
The variety of possible combinations of kinases engaged by the initial stimulus, the presence of parallel or converging signaling, the intervention of specific scaffold proteins,1 and the magnitude and duration of signaling determine the final outcome.
6.1 Main Features of MAPK Signaling
Cells possess multiple MAPK modules that are constituted by at least 3 tiers. Nevertheless, signaling MAPK network architecture can change owing to the modular design of MAPK axes that can acquire new partners [534].
Final mediators include many MAPK enzymes grouped into 3 main families: (1) extracellular signal-regulated kinases with the major types ERK1 (or MAPK3) and ERK2 (MAPK1 or MAPK2) as well as other components ERK4 (a.k.a. ERK5, MAPK6, and MAPK7) and ERK7 (ERK8 or MAPK15);2 (2) Jun N-terminal kinases (JNK1–JNK3, or MAPK8–MAPK10); and (3) P38MAPK enzymes (P38MAPKα–P38MAPKδ, or MAPK14 and MAPK11–MAPK13, respectively).
6.1.1 Basic Three-Tiered MAPK Module
The prototypical mitogen-activated protein kinase module comprises, at least, 3 types of kinases: (1) upstream Ser/Thr kinase; (2) middle dual-specificity kinase; and (3) downstream Ser/Thr kinase [536]. The 3-tiered kinase modules are constituted by different members and have distinct activation mechanisms.
At each tier, a kinase activates the kinase of the next tier. Furthermore, full activation of the kinase requires double phosphorylation. The MAPK enzymes are activated by concomitant Tyr and Thr phosphorylation of a Thr–X–Tyr (X: any amino acid) phosphoacceptor motif in the activation loop of the kinase domain [267].3
The upstream kinase and a phosphatase control the phosphorylation state of the 2 sites of the target kinase. The upstream kinase can be dephosphorylated (deactivated) by a phosphatase and thus must be reactivated with a given time scale to process the second phosphorylation.
6.1.2 Double Phosphorylation Cycle
The double phosphorylation cycle can either rely on a processive or distributive process. In a distributive mechanism, the kinase is released from its substrate after it has modified the first site. Afterward, it can rebind and modify the second site. In a processive mechanism, the enzyme remains bound to its substrate during the entire processing, from first to second phosphorylation. A distributive mechanism can generate an ultrasensitive response because the concentration of the fully-activated kinase depends on the upstream kinase concentration [538].
6.1.3 MAPK Pathway
A typical signaling pathway involves receptor Tyr kinases that are activated by ligand binding (leading to possible receptor dimerization and autophosphorylation) and then recruit signaling proteins. Recruited proteins are phosphorylated (activated) and then interact with other signaling mediators. In addition, multiple MAPK pathways, together with the nuclear factor-κB pathway, are targeted by environmental stress and inflammatory cytokines, especially those of the tumor-necrosis factor superfamily.
Mitogen-activated protein kinases are substrates of upstream kinases of the MAPK cascades, i.e., MAPK kinases (MAPKK, MAP2K, MEK, or MKK) and MAPK kinase kinases (MAPKKK, MAP3K, MEKK, or MKKK; Table 6.1).4 The latter can be activated by MAPKKK kinase (MAPKKKK or MAP4K) that can be considered as the transducers of 3-tier MAPK modules (Table 6.2). They tether to the plasma membrane or other signaling mediators.
Table 6.1
Mitogen-activated protein kinase (MAPK) modules and their stimuli. Each mitogen-activated protein kinase cascade consists of a main triple-decker part with a MAP kinase kinase kinase (MAP3K), a MAP kinase kinase (MAP2K), and a MAP kinase (MAPK). Enzymes of the MAP4K set comprise various enzymes (GCK: germinal center kinase; HPK: hematopoietic protein kinase; and PAK: p21-activated kinase). The MAP3K set also include many members (ASK: apoptosis signal-regulating kinase; MLK: mixed lineage kinase; TAK: transforming growth factor-β-activated kinase; and TPL1: tumor progression locus-1). Pathway effectors differ according to the stimulus type. MAP2K1 and MAP2K2 are dual-specificity kinase that phosphorylate ERK1 (Thr183) and ERK2 (Tyr185), respectively; MAP2K3 (phosphorylated at Ser189 and Thr193) targets P38MAPK (at Thr180 and Tyr182); MAP2K4 activates both P38MAPKs and Jun N-terminal kinases (JNK) that are called stress-activated protein kinase (SAPK); MAP2K5, MAP2K6, and MAP2K7 stimulate ERK5, P38MAPK, and JNK, respectively. Proteins of the MAPK set can translocate to the nucleus and phosphorylate transcription factors (ATF: activating transcription factor; CHoP: CCAAT/enhancer-binding protein homologous protein; CREB: cAMP-responsive element-binding protein; ELk: member of DNA-binding ETS [E26] domain transcription factor family; and MEF: myocyte enhancer factor).
Stimuli | Growth factors | Growth factors, stresses |
---|---|---|
Pro-inflammatory cytokines, TNFα | ||
Small GTPases | Ras, Rap | Rho, Rac, CDC42 |
MAP4K | GCK, HPK1, PAK1 | |
MAP3K | Raf | MAP3K1/4, JN3K (ASK, MLK2/3, TAK) |
MAP2K | MAP2K1/2/5 | MAP2K |
MAPK | ERK1/2/5 | JNK, P38MAPK |
Transcription | TCF, MEF2C | ATF2, CREB, CHoP, MEF2C (P38MAPK) |
factors | ATF2, ELk1, Jun (JNK) |
Table 6.2
Mitogen-activated protein kinase kinase kinase kinases (GCK: germinal center kinase; GCKR: GCK-related kinase; GLK: GCK-like kinase: HGK: HPK/GCK-like kinase; HPK: hematopoietic progenitor kinase; KHS: kinase homologous to Ste20 protein kinase; MinK: misshapen-like kinase; NIK: NCK-interacting kinase [not NFκB-inducing kinase (NIK or MAP3K14)]).
Type | Other aliases |
---|---|
MAP4K1 | HPK1 |
MAP4K2 | GCK, BL44, Rab8IP |
MAP4K3 | GLK |
MAP4K4 | NIK, HGK |
MAP4K5 | GCKR, KHS, KHS1 |
MAP4K6 | MinK |
The MAPK modules that are constituted of affectors such as MAP4Ks, a set of effector kinases (MAP3Ks, MAP2Ks, and MAPKs), and scaffold proteins, target various types of proteins (Table 6.3).
Table 6.3
Target enzymes of MAPK modules (Source: [10]; cPLA2: cytoplasmic phospholipase-A2; MAPKAPK: MAPK-activated protein kinase; MSK: mitogen- and stress-activated protein kinase; PTPR: receptor-type protein Tyr phosphatase).
Type | Examples |
---|---|
Kinase | MAPKAPKs, MSK1/2 |
Phosphatase | PTPRh |
Phospholipase | cPLA2 |
6.1.4 MAPK Stimuli
Diverse stimuli operate via various types of receptors to initiate MAPK-mediated signaling using multiple activators and inhibitors upstream from MAPK modules: (1) hormones and growth factors that act via receptor Tyr kinases (Vol. 3 – Chap. 8. Receptor Kinases) and cytokine receptors, but transforming growth factor-β-related proteins target receptor Ser/Thr kinases; (2) vasoactive peptides that binds to G-protein-coupled receptors; (3) inflammatory cytokines of the tumor-necrosis factor superfamily; and (4) environmental stresses (e.g., ischemic injury, osmotic shock, and ionizing radiation).
6.1.5 MAPK Cascade Regulation
The MAPK cascades are composed of specific tiers of signal transmission from environmental stimuli (Fig. 6.1, Table 6.4). There are up to 6 activation levels in the cytosol and nucleus that encompass the specific 3-tiered MAP3K–MAP2K–MAPK cascade. Regulation of MAP3K is achieved by membrane recruitment, homo-oligomerization, often formation of a proteic complex with regulators, and phosphorylation.


Fig. 6.1
Mitogen-activated protein kinase cascades in cells correspond to a reaction cascade with several steps that include the 3-tiered MAP3K–MAP2K–MAPK module (Source: [539]). Enzyme MAP3K is the usual entry node of MAPK cascades, although MAP3Ks can sometimes be stimulated by MAP4K proteins. Mechanical stresses, particularly in blood vessel wall, stimulate mediators of the MAPK cascade. Effectors of the MAPK cascade lead to gene transcription for adaptation and, if necessary when subjected to long-duration high-magnitude forces, for tissue remodeling.
Table 6.4
Extracellular stimuli activate MAPK pathways via small GTPases. Activated MAP3Ks (e.g., Raf, apoptosis-related kinases [ASK], mixed lineage kinases [MLK], TGFβ-activated kinase [TAK], etc.) phosphorylate MAP2Ks on 2 serine residues, which in turn phosphorylate MAPKs, extracellular signal-regulated kinase (ERK), Jun N-terminal kinase (JNK), and P38MAPK on both threonine and tyrosine residues. Activated MAPKs can translocate to the nucleus and phosphorylate transcription factors (ternary complex factor TCF, activator protein AP1 with JUN, and activating transcription factor ATF2). TCF forms a complex with serum response factor (SRF).
Cascade 1 | Cascade 2 | Cascade 3 | |
---|---|---|---|
Tier 1 | Ras | MAP3K | TAK |
Tier 2 | MAP2K1/2 | MAP2K4/7 | MAP2K3/6 |
Tier 3 | ERK | JNK | P38MAPK |
Transcription factor | SRF–TCF | SRF–TCF | SRF–TCF |
AP1 | ATF2 |
The MAPK pathway contains autoregulatory loops. In particular, the ERK axis can regulate the production of MAPK phosphatase MKP1 (Table 6.5) that trigger a negative feedback loop.
Table 6.5
Phosphatases of the MAPK modules (Source: [10]; DUSP: dual-specificity phosphatase; PTPn: protein Tyr phosphatase, non-receptor).
Category | Types |
---|---|
DUSP | DUSP2/3/5/7/8 |
MAPK phosphatases | MKP1–MKP5 |
PTPn | PTPn5 |
6.1.6 Auto-Inhibition
Auto-inhibition is a functioning mode of protein kinase. The catalytic domain binds ATP and substrates for phosphoryl transfer. They change their activation region into an auto-inhibitory module [540]. The activation region must unlock from another molecular segment to tether ATP.
6.1.7 Scaffold Proteins
Signaling pathway organization is mediated by scaffold proteins (Table 6.6). Scaffold proteins couple MAPK components to upstream activators. Signaling efficiency of MAPK modules results, at least partly, from scaffolds that organize MAPK signaling components into functional elements to activate specific MAPK pathways. Scaffold proteins of MAPK signaling also allow specific signal transduction, as they bind to selected MAPK constituents. They are able to segregate adequate axes among MAPK modules and maintain signal transduction specificity. Furthermore, they protect signaling components from undesirable activation by other signaling molecules. They also enable a coordinated activation of MAPK modules in response to given stimuli. Some MAPK interactors can couple constituents of different MAPK modules. Elements of MAPK modules can indeed be targeted by several activators and, in turn, connect to several effectors.
Table 6.6
Scaffold proteins of the mitogen-activated protein kinases (CNK2: CNK homolog protein; CnKSR: connector enhancer of kinase suppressor of Ras; JIP: JNK-interacting protein; KSR: kinase suppressor of Ras; LAMTOR: late endosomal/lysosomal adaptor, MAPK and TOR activator; maguin: membrane-associated guanylate kinase-interacting protein; MAPKAP1: MAPK-associated protein; MP: MEK-binding partner; SIN: stress-activated MAPK (SAPK)-interacting protein [a.k.a. target of rapamycin complex TORC2 subunit]; SKRP: stress-activated protein kinase (SAPK) pathway-regulating phosphatase; TAB: TAK1-binding protein (MAP3K7IP1–MAP3K7IP3; MAP3K7IP1 is a MAP3K7 activator, whereas MAP3K7IP2 and -3 are adaptors). The dual-specificity phosphatase DUSP7 can also function as a scaffold. The MAP3K12 binding inhibitory protein MAP3K12IP1 is the MAPK upstream kinase (MUK)-binding inhibitory protein (MBIP).
Type | Other aliases |
---|---|
β-Arrestin-2 | |
CnKSR2 | CNK2, maguin |
DUSP7 | MKPX |
DUSP19 | SKRP1, DSP3lMW |
KSR1 | RSU2 |
KSR2 | |
MAP2K1IP1 | LAMTOR3, MAPKSP1, MAPBP, MP1, ragulator-3 |
MAP3K7IP1 | TAB1 |
MAP3K7IP2 | TAB2 |
MAP3K7IP3 | TAB3 |
MAPK8IP1 | JIP1 |
MAPK8IP2 | JIP2 |
MAPK8IP3 | JIP3 |
MAPK8IP4 | JIP4 |
MAPKAP1 | MIP1, SIN1 |
Stress-activated protein kinase-associated proteins, or Jun N-terminal kinase-interacting proteins, operate as scaffold proteins for JNK cascades. Jun N-terminal kinase-associated leucine zipper protein (JLP; JIP4 or MAPK8IP4) is a scaffold for the JNK and P38MAPK signaling cascades that also acts as a binding partner for MAX transcription factor. It interacts with several proteins, such as Gα13 protein, kinesin light chain-1, and cell-surface receptor-like glycoprotein Cell adhesion molecule (CAM)-related downregulated by oncogenes CDO of the immunoglobulin–fibronectin-3 repeat-containing protein family in myocyte precursors.
Connector enhancer of kinase suppressor of Ras CnKSR25 is an enzyme that also operates as a scaffold protein to mediate MAPK signals downstream from Ras GTPase. It is stimulated by vitamin-D. The CnKSR2–KSR2 complex may enable crosstalk between the MAPK, PI3K, and insulin pathways [541]. It can complex with cRaf, RalA, MAP2K1, MAP2K2, PI3K, and CDK4 kinases, as well as PP2 phosphatase.
6.1.8 Transcription Factor Substrates of MAPK Modules
Activated MAPKs can translocate to the nucleus to phosphorylate a number of transcription factors, such as members of the ternary complex factor (TCF) family, which associate with serum response factor and target the FOS gene, and components of the Activator protein-1 complexes, such as Jun and activating transcription factor ATF2 (Table 6.7).
Table 6.7
Target transcription factors of MAPK modules (ATF: activating transcription factor; ELk: ETS-like transcription factor; ETS: E26 (E-twenty six) transcription factor family; MEF: myocyte-enhancer factor; SRF: serum response factor; TCF: ternary complex factor [ELk1, ELk3, ELk4]). Transcription ETS factors, or ternary transcription factors, complex with DNA and SRF factor.
Type | Components |
---|---|
(versions) | |
Activator protein-1 | ATF2, Jun, JunB, JunD |
ETS | ETS1, ELk1 |
SRF–TCF | |
MEF2 | MEF2a–MEF2d versions |
The Ras–MAPK signaling activates transcription factors of the ETS family. Phosphorylation improves the affinity (34-fold) of ETS1 for transcriptional coactivators CREB-binding protein and P300 that also serve as histone acetyltransferases, polyubiquitin ligases, and adaptors [542]. Ras-responsive elements include the serum-response-element of promoter of the FOS gene, tandem ETS, or ETS–AP1 composite sites associated with ETS1 and ETS2.
6.1.9 Subcellular Compartmentation
Mitogen-activated protein kinases are linked to various organelles, such as microtubules, endosomes, endoplasmic reticulum, and actin cytoskeleton. Active MAPKs often translocate from the cytosol to the nucleus to phosphorylate nuclear targets. Enzymes of the MAP2K set can carry MAPK from the cytoplasm to the nucleus and inversely.
6.2 MAPK Modules in Cell Metabolism
Signaling based on MAPK modules is involved in almost all of the changes in the extra- or intracellular medium that affect the cell metabolism. Metabolic adaptation actually relies on MAPK-dependent signal transduction. On the other hand, inappropriate MAPK signaling contributes to the development of metabolic syndrome [535].
Hormones (e.g., glucagon and insulin), growth factors (e.g., IGF1 and EGF), cytokines (e.g., TNFα), and environmental stress prime MAPK signaling (Table 6.8). Activated MAPKs directly or indirectly via MAPKAPK1 proteins (or P90RSK; MAPKAPK1a–MAPKAPK1c),6 MAPKAPK2 (or MK2), P90RSK subfamily enzymes MSK1 and MSK2),7 MAPK-interacting protein Ser/Thr kinases MKnK18 and MKnK2,9 regulate numerous metabolic agents.
Table 6.8
Regulation of cell metabolism by MAPK modules and their effector kinases triggered by epithelial growth factor (EGFR), glucagon (GcgR), insulin (IR) and insulin growth factor (IGF1R), and tumor-necrosis factor (TNFR) receptors (Source: [535] ATF: activating transcription factor; CREB: cAMP response element-binding protein; C/EBP: CCAAT/enhancer binding protein; eIF: eukaryotic translation initiation factor; Fox: forkhead box; GR: glucocorticoid receptor; GSK: glycogen synthase kinase; HIF: hypoxia-inducible factor; HSL: hormone-sensitive lipase; MAPKAPK: MAPK-activated protein kinase; MKnK: MAPK-interacting kinase (or MnK); MSK, mitogen- and stress-activated kinase (or 90-kDa ribosomal protein S6 kinase); PGC: PPARγ coactivator; PPAR: peroxisome proliferator-activated receptor; SREBP: sterol regulatory element-binding protein; TORC: target of rapamycin complex; TSC: tuberous sclerosis complex).
Effect | Effectors (and their activators) |
---|---|
Gluconeogenesis | C/EBPα (ERK, P38MAPK), CREB (MSK), |
FoxO1 (ERK, P38MAPK), | |
GR (ERK, JNK, P38MAPK), | |
PPARα (ERK, P38MAPK), PGC1α (P38MAPK) | |
Glycogen synthesis | GSK3 (MAPKAPK1, MSK) |
Lipid homeostasis | C/EBPα (ERK, P38MAPK), |
HIF1α (ERK, P38MAPK), | |
SREBP1a/2 (ERK) | |
Lipolysis | HSL (ERK), perilipin (ERK, JNK) |
Protein synthesis | eIF4b (MAPKAPK1), eIF4e (MKnK), |
TORC1 (ERK), TSC1/2 (ERK, MAPKAPK1) | |
Adipocyte differentiation | ATF2 (P38MAPK), C/EBPα (ERK, P38MAPK), |
C/EBPβ (P38MAPK), PPARγ (ERK, JNK) |
6.2.1 Insulin and Glucagon Signaling
Insulin activates not only the PI3K–PKB pathway, but also MAPK modules [535]. Upon binding of the insulin receptor substrate (IRS) or SHC adaptor to the activated insulin receptor and subsequent phosphorylation, GRB2 adaptor is recruited and, then, associates with SOS guanine nucleotide-exchange factor to activate the Ras–Raf–ERK pathway. In addition, insulin can activate P38MAPK and JNK, involving in the latter case, the small CDC42 GTPase and PI3K regulatory subunit P85 (P85PI3K) to activate MAP2K4 enzyme.
Upon insulin stimulation, inhibitory phosphorylation of glycogen synthase kinase GSK3 by P90RSK enzymes, especially those of the MAPKAPK1 group, which are substrates of ERK1 and ERK2, as well as by PKB, and activation of PP1 stimulate glycogen synthase [535].
Once it is activated by insulin, PKB phosphorylates FoxO1 transcription factor, thereby leading to its nuclear export and stopping the transcription of enzymes of gluconeogenesis, lipid catabolism, and mitochondrial function [535]. Moreover, FoxO1 is phosphorylated by ERK1, ERK2, and p38MAPKs.
Kinases P38MAPKα and P38MAPKβ may be involved in glucose uptake using glucose transporter GluT4 in response to insulin. Insulin also stimulates protein synthesis and may act via MAPK modules.
Transcription factors of the SREBP family (SREBP1a, SREBP1c, and SREBP2) are major regulators of insulin-primed lipogenesis.10 Insulin-activated ERK1 and ERK2 phosphorylates SREBP2 and possibly SREBP1a factor [535].
On the other hand, insulin suppresses gluconeogenesis and lipid catabolism in the liver, whereas glucagon and glucocorticoids have the opposite effect during fasting.11 Glucagon enhances the production of PPARγ and PGC1 via the cAMP pathway and CREB transcription factor [535].12 Enzymes of the P38MAPK set stimulate the glucagon-mediated fasting response in the liver, as they phosphorylates various key agents.13
6.2.2 MAPKs in Adipocytes
Adipose tissue participates in nutrient and energy homeostasis (Vol. 2 – Chap. 1. Remote Control Cells). In white adipose tissue, when energy is needed, triacylglycerides are hydrolyzed into free fatty acids and glycerol by 3 lipase types. Upon TNFα stimulation, activated JNKs, ERK1 and ERK2 increase basal lipolysis.
These members of the MAPK superfamily indeed repress perilipin-1 synthesis.14 Moreover, ERK1 and ERK2 phosphorylate (activate) hormone-sensitive lipase.
Both ERK1 and ERK2 contribute to adipocyte differentiation. Temporal control of ERK activity is necessary during adipogenesis, ERK1 and ERK2 being activated early in cellular differentiation. Factor PPARγ (or nuclear receptor NR1c3), the master regulator of adipocyte differentiation, is phosphorylated (inhibited) by ERK and JNK kinases. Adipocyte differentiation is also supported by P38MAPKs that phosphorylate C/EBPβ and target activating transcription factor ATF2 that promotes PPARγ transcription.
6.2.3 MAPKs in Metabolic Syndrome
Excessive diet intake causes lipid accumulation and various metabolic imbalances that lead to insulin resistance, dyslipidemia, inflammation, and hypertension (Vol. 6 – Chap. 7. Vascular Diseases). Metabolic syndrome is a major risk factor for type-2 diabetes mellitus and atherosclerosis.
Enzymes of the MAPK set are involved in the development of insulin resistance. In particular, JNK1 contributes to increase adipose mass under hypercaloric conditions [535]. Kinases of the JNK subset can inhibit insulin signaling, as they phosphorylate IRS1 (Ser307) in insulin-sensitive cell types other than hepatocytes.15 In hepatocytes, insulin stimulation indeed leads to IRS1 phosphorylation (Ser307).
Kinase ERK1 interacts with and is inhibited by the adaptor IGF2-binding protein IGF2BP2 [535]. Protein ERK1 is required in the early stage of adipogenesis, but needs to be inactivated to enable PPARγ activity in late stage of adipogenesis.
In the pancreas, P38MAPKδ prevents PKD1 activity that regulates insulin secretion and β-cell survival. In addition, under stress, JNK activation that relies on the scaffold JNK-interacting protein MAPK8IP1 can provoke failure of β-cell functioning [535].
6.3 Mitogen-Activated Protein Kinase Kinase Kinase Kinases
Many mitogen-activated protein kinase kinase kinase kinases (MAP4K) belong to the Sterile-20-like kinase superfamily (Sect. 5.1), more precisely to the germinal center kinase (GCK) family.16 Members of the GCK family (MAP4Ks) indeed include: MAP4K1 (or hematopoietic progenitor kinase HPK1); MAP4K2 (or germinal center kinase [GCK]); MAP4K3 (or GCK-like kinase [GLK]); MAP4K4 (or non-catalytic region of Tyr kinase adaptor [NCK]-interacting kinase [NIK]); MAP4K5 (or GCK-related kinase [GCKR]); and MAP4K6 (or misshapen-like [MinK or MinK1] NIK-related kinase; Sect. 5.1.2).
The GCK subfamily 1 comprises MAP4K1 to MAP4K3 and MAP4K5, whereas the GCK subfamily 4 contains MAP4K4 and MAP4K6 (MinK), as well as TRAF2- and NCK-interacting kinase (TNIK) and NIK-related kinase (NRK). Members of the GCK subfamily 4 within the GCK family are slightly divergent from subfamily-1 kinases.
Enzyme MAP4K2 is a specific activator of Jun N-terminal kinase, as it does not activate ERKs, P38MAPKs, and nuclear factor-κB. This stress-activated kinase that has a basal activity is activated in vivo by signaling affectors.
Enzyme MAP4K5 is also a specific activator of Jun N-terminal kinase that has a much smaller basal activity than MAP4K2. It is another effector of tumor-necrosis factor. Enzyme MAP4K3 is a third GCK subfamily-1 member that is also a selective JNK activator stimulated by tumor-necrosis factor.
Enzyme MAP4K1 is more distantly related to MAP4K2 than MAP4K5 and MAP4K3 enzymes. It interacts with MAP3K11 (or mixed lineage kinase MLK3). It stimulates strongly JNK kinases and weakly P38MAPK enzymes. It is recruited by adaptors GRB2, CRK, and CRKL to the plasma membrane and plasmalemmal receptors such as EGFR. Its activation of JNKs is indeed primed by mitogens such as EGF via their cognate receptor Tyr kinases.
Enzyme MAP4K4, like its close relatives NRK and TNIK, is the kinase most distantly related to MAP4K2 protein. However, like MAP4K2, it is activated by TNF and strongly and specifically activates JNKs. Adaptor NCK couples MAP4K4 to the EPH family of receptor Tyr kinases.
Enzyme MAP4K6, like MAP4K4, is an effector for TNFR-associated factors (TRAF). Enzyme TNIK, another MAP4K, provokes a potent and specific JNK activation that also can interact with NCK adaptor and TRAF2 protein.
Among the group-2 GCKs (Sect. 5.1.4), high levels of STK4 (MST1 or KRS2) can activate JNK and P38MAPKs [267]. Members of another group of GCKs (GCK subfamily 8; Sect. 5.1.10), thousand and one kinases (TAOK1–TAOK3) serve as MAP3Ks (MAP3K16–MAP3K18), and not upstream affectors such as MAP4Ks, that can activate certain MAPK pathways (Sect. 6.5.7).17
6.4 Other Regulators of the MAPK Module
6.4.1 Small GTPases of the Ras Hyperfamily
Monomeric GTPases of the RAS hyperfamily are especially involved in the activation of the ERK pathway mainly via cRaf kinase. They are stimulated by guanine nucleotide-exchange factors (GEF) and inactivated by GTPase-activating proteins (GAP). Activation of cRaf by Ras follows its translocation to the plasma membrane and leads to its phosphorylation by recruited cytosolic Tyr protein kinases such as Src or Ser/Thr protein kinases such as p21-activated kinase PAK3 and homo-oligomerization.
In addition, small GTPase Rac1 (but not CDC42, Ras, or Rho) interacts with SH3 domain-containing ring finger protein SH3RF1 (or plenty of SH3s [POSH]) that triggers nuclear translocation of NFκB and activates Jun N-terminal kinases. Small GTPase CDC42 (but not Rac1) can target MAP3K10 (or MLK2) and MAP3K11 (or MLK3) via adaptor SH3RF1. Furthermore, PAKs as well as MAP3K1 and MAP3K4 can interact with both Rac1 and CDC42 [267].
6.4.2 Heterotrimeric G Proteins
Heterotrimeric G proteins can couple to Jun N-terminal kinases via monomeric Ras GTPase or cytosolic Tyr kinases. Bruton’s Tyr kinase is activated by G12 subunit to stimulate Jun N-terminal kinases [267]. Subunits Gq and free Gβγ can activate phospholipase-Cβ, thereby causing calcium ion influx that stimulates FAK2 kinase. The latter, together with Src, transmits signals from G proteins to extracellular signal-regulated protein kinases [267].
6.5 Mitogen-Activated Protein Kinase Kinase Kinases
Mitogen-activated protein kinase kinase kinases (MAP3K; Table 6.9) constitute an epifamily that comprises diverse families of protein kinases [545, 546] (Tables 6.10 to 6.13): (1) ASK (apoptosis signal-regulating kinase) with ASK1 and ASK2 members (i.e., MAP3K5–MAP3K6); (2) MEKK (MAP/ERK kinase kinase, i.e., MEKK1–MEKK4, or MAP3K1–MAP3K4); (3) MLK (mixed lineage kinase, i.e., MAP3K9–MAP3K13); (4) Mos (uterus Moloney sarcoma viral v-Mos proto-oncogene product); (5) Raf (the first RAF gene corresponds to acute transforming retrovirus oncogene homologous to the SRC family of sarcoma virus-related proto-oncogenes [v-Raf]); (6) TAK1 (transforming growth factor-β-activated kinase-1 or MAP3K7); (7) TAOK (thousand and one amino acid kinases, i.e., TAOK1, MAP3K16, MARKK, PSK2, or KFCb; TAOK2, MAP3K17, PSK1, or KFCc; and TAOK3, MAP3K18, DPK, JIK, or KFCa); and (8) TPL2 (tumor progression locus-2 or MAP3K8). Many MAP3Ks belong to the Sterile-20-like kinase superfamily. In fact, the GCK subfamily 8 (Sect. 5.1.10) of the Sterile-20-like kinase superfamily is constituted by members of the TAOK family.
Table 6.9
Mitogen-activated protein kinase kinase kinases (ASK: apoptosis-signal-regulating kinase; DLK: dual leucine zipper-bearing kinase; MEKK: mitogen–extracellular signal-regulated kinase kinase; MLK: mixed lineage kinase; MTK: MAP three kinase; TAK: TGFβ-activated kinase; TAOK: thousand and one amino acid kinase; TPL: tumor progression locus-2).
Type | Other aliases |
---|---|
aRaf | |
bRaf | |
cRaf | Raf1 |
MAP3K1 | MEKK1 |
MAP3K2 | MEKK2 |
MAP3K3 | MEKK3 |
MAP3K4 | MEKK4, MTK1 |
MAP3K5 | MEKK5, ASK1 |
MAP3K6 | MEKK6, ASK2 |
MAP3K7 | MEKK7, TAK1 |
MAP3K8 | MEKK8, Cot, TPL2 |
MAP3K9 | MEKK9, MLK1 |
MAP3K10 | MEKK10, MLK2, MST |
MAP3K11 | MEKK11, MLK3, SPRK, PTK1 |
MAP3K12 | MEKK12, DLK, MUK, ZPK |
MAP3K13 | MEKK13, LZK |
MAP3K14 | NIK, HS, HSNIK |
MAP3K15 | MLK7, ZAK, MRK, MLTK |
MAP3K16 | TAOK1, MARKK, PSK2, KFCb |
MAP3K17 | TAOK2, PSK, PSK1, TAO1, TAO2, KFCc |
MAP3K18 | TAOK3, DPK, JIK, KFCa |
Table 6.10
Features of MAP3Ks. (Part 1) Raf and Mos (Source: Sigma-RBI eHandbook; BAG: BCL2-associated athanogene; CNKSR: connector enhancer of kinase suppressor of Ras (or CNK); KSR: kinase suppressor of Ras; MyoD: Myogenic differentiation transcription factor; MyT: myelin transcription factor; PEBP: phosphatidylethanolamine-binding protein [or RKIP]; RIP: receptor-interacting protein; SHOC: suppressor of clear homolog protein [SOC2 or SUR8]). All MAPK signal transduction axes correspond to 3-kinase (MAP3K–MAP2K–MAPK) cascades. MAPK signaling modules mediate cellular responses to hormones, growth factors, cytokines, and environmental stresses.
Distribution | Binding | Activators | Affectors | Effectors | Function |
---|---|---|---|---|---|
Location | partners | ||||
Raf MAP3Ks: a-, b-, and cRaf | |||||
Ubiquitous | Pax, | Growth | Ras, | MAP2K1/2 | Cell growth |
Cytosol | KSR1/2, | factors | Rap, | ||
BAG1, | PAK | ||||
CNKSR, | |||||
PEBP1, | |||||
SHOC2, | |||||
14-3-3 | |||||
Mos MAP3K | |||||
Germ cell | Progesterone | CDK1 | MAP2K1/2, | Oocyte | |
Cytosol | Growth | MyT1, | maturation | ||
factors | MyoD1 |
Table 6.11
Features of MAP3Ks. (Part 2) MEKK (Source: Sigma-RBI eHandbook; GADD: growth arrest and DNA-damage protein; JLP: JNK-associated leucine zipper protein (or SpAg9); JNKBP: JNK-binding protein; JSAP: JNK/SAPK-associated protein; LAd: LCK-associated adaptor (Rlk- and Itk-binding protein RIBP); MIP1: MAP3K2-interacting protein; OsM: oncostatin-M; TRAF: tumor-necrosis factor receptor-associated factor). All MAPK signal transduction axes correspond to 3-kinase (MAP3K–MAP2K–MAPK) cascades. MAPK signaling modules mediate cellular responses to hormones, growth factors, cytokines, and environmental stresses.
Distribution | Binding | Activators | Affectors | Effectors | Function |
---|---|---|---|---|---|
Location | partners | ||||
MEKKs: MAP3K1–MAP3K4 | |||||
Ubiquitous | JNKBP, | Growth | Rac1, | MAP2K1–7 | Stress |
Cytosol | JSAP, | factors, | Ras, | IKK | response, |
JLP, | stress | CDC42, | development | ||
GADD45, | MAP4K1/2 | ||||
LAd, | |||||
MIP1, | |||||
OsM, | |||||
TRAF, | |||||
14-3-3 |
Table 6.12
Features of MAP3Ks. (Part 3) ASK and MLK (Sources: Sigma-RBI eHandbook and BioGRID; Develop.: development; CKI: cyclin-dependent kinase inhibitor [CKI1a; a.k.a. p21, Cip1, and Waf1]; DAXX: death-associated protein [DAP6 or BING2]; GADD: growth arrest and DNA-damage protein; GlnRS: glutaminyl-tRNA synthase; GST: glutathione S-transferase; IRE1: inositol-requiring endoplasmic reticulum-resident transmembrane kinase/endoribonuclease (or endoplasmic reticulum-to-nucleus signaling ERN1); MBIP: MUK-binding inhibitory protein (MAP3K12IP1); SH3RF: SH3 domain-containing ring finger protein (SH3RF1 or plenty of SH3s [POSH]); TRAF: tumor-necrosis factor receptor-associated factor).
Distribution | Binding | Activators | Affectors | Effectors | Function |
---|---|---|---|---|---|
Location | partners | ||||
ASKs: ASK1/MAP3K5 and ASK2/MAP3K6 | |||||
Ubiquitous | β-Arrestin-2, | Stress | IRE1, | MAP2K3/4, | Stress |
Cytosol | Thioredoxin, | Calcium | CamK2 | MAP2K6/7 | response |
MAP3K5–7, | Neuron | ||||
GlnRS, PP5, | apoptosis | ||||
eIF2αK2, | Heart | ||||
CDC25a, | function | ||||
DUSP19, | |||||
DAXX, | |||||
GSTμ1, | |||||
TRAF1–3/5/6, | |||||
Hsp70-1a, | |||||
CKI1a, cRaf, | |||||
JIP3, | |||||
GADD45b, | |||||
14-3-3 | |||||
MLKs: MLK1–MLK4, MLK7, DLK, LZK | |||||
Ubiquitous | JIP1–3, | Stress | MAP4K1, | MAP2K3/4, | Stress |
Cytosol | MBIP, | GF | CDC42, | MAP2K6/7 | response |
SH3RF1, | Cytokines | Rac1, | Develop. | ||
14-3-3 | PAK |
Table 6.13
Features of MAP3Ks. (Part 4) TAK1, TAOK, and TPL2 (Sources: Sigma-RBI eHandbook and BioGRID; Inflam.: inflammation; IKK: inhibitor kinase of nuclear factor-κB; HGS: hepatocyte growth factor-regulated tyrosine kinase substrate; HIPK: homeodomain-interacting protein kinase; IRE1: inositol-requiring endoplasmic reticulum-resident transmembrane kinase/endoribonuclease [or endoplasmic reticulum-to-nucleus signaling ERN1]; RKIP: Raf kinase inhibitor protein [phosphatidylethanolamine-binding protein (PBP) or osteoclast differentiation factor receptor (ODFR)]; IL17Rd: interleukin-17 receptor-D [or similar expression to FGF (SEF1)]; TAB: TAK1-binding protein [MAP3K7IP1–MAP3K7IP3]; TNFAIP: tumor-necrosis factor-α-interacting protein [or A20-binding inhibitor of nuclear factor-κB (ABIN)]; TIRAP: Toll-interleukin-1 receptor domain-containing adaptor protein; TRAF: tumor-necrosis factor receptor-associated factor).
Distribution | Binding | Activators | Affectors | Effectors | Function |
---|---|---|---|---|---|
Location | partners | ||||
TAK1 | |||||
Ubiquitous | SMAD3/7, | TGFβ | MAP4K1/4, | MAP2K3/4, | Stress |
Cytosol | RKIP, | Wnt | Ubiquitin | MAP2K6, | response |
HGS, IL17Rd, | Stress | IKK, | |||
MAP3K5/7/14, | Cytokines | HIPK2 | |||
TGFβR1, | |||||
TAB1–3 | |||||
MyD88, TIRAP, | |||||
TRAF2/6, | |||||
TAOK MAP3Ks: TAOK1/MAP3K16–TAOK3/MAP3K18 | |||||
Ubiquitous | Tubulin, | Osmotic | IRE1 | MAP2K3/4, | Stress |
Cytosol | TRAF2 | stress | MAP2K6/7 | response | |
TPL2/MAP3K8 | |||||
Ubiquitous | NFκB, | Cytokines, | MAP4K4 | MAP2K1/2, | Inflam. |
Cytosol | PKB, STK4, | LPS | MAP2K4–6 | ||
TRAF2, | IKK | ||||
TNFAIP2, | |||||
MAP3K14, | |||||
KSR2 |
Most MAP3K enzymes are regulated, at least partly, by 4 processes: oligomerization (e.g., cRaf, MAP3K1, MAP3K5, and MAP3K11), adaptor binding, membrane translocation, and phosphorylation and trans-autophosphorylation. Regulatory domains of MAP3Ks interact with upstream regulators, such as Rho and Ras GTPases. Activated MAP3K phosphorylates (activates) one or several MAP2Ks. Many MAP3Ks also activate the IKK–NFκB pathway.
The MAPK pathways can be subdivided into those that predominantly respond to either mitogens to regulate cell growth and differentiation (e.g., extracellular signal-regulated kinases ERK1 and ERK2) or stress (Jun N-terminal kinases and P38MAPKs) to control cell apoptosis and immune response (Table 6.14). The ERK5 pathway responds to both growth stimuli and some stresses. Activity of MAP3K is regulated by various mechanisms, such as phosphorylation and proteolysis as well as interactions with small GTPases and other regulators and linkage to scaffold proteins.
Table 6.14
Types of MAP2Ks and MAPKs targeted by MAP3Ks.
MAP3K | Alias | MAP2K | ERK1/2 | ERK4 | JNK | P38MAPK |
---|---|---|---|---|---|---|
(main) | ||||||
MAP3K1 | MEKK1 | MAP2K4/7 | + | + | + | |
MAP3K2 | MEKK2 | MAP2K1/4 | + | + | + | + |
MAP3K3 | MEKK3 | MAP2K1/4 | + | + | + | + |
MAP3K4 | MEKK4 | MAP2K3/4/6 | + | + | ||
MAP3K5 | ASK1 | MAP2K3/4/6 | + | + | ||
MAP3K6 | ASK2 | MAP2K3/4/6 | + | + | ||
MAP3K7 | TAK1 | MAP2K3/4/6 | + | + | ||
MAP3K8 | TPL2 | MAP2K1/4 | + | + | + | |
MAP3K9 | MLK1 | + | + | + | + | |
MAP3K10 | MLK2 | MAP2K4/7 | + | + | + | + |
MAP3K11 | MLK3 | MAP2K4/7 | + | + | + | + |
MAP3K12 | DLK | MAP2K4/7 | + | + | + | |
MAP3K13 | LZK | + | + | + | ||
MAP3K14 | HSNIK | |||||
MAP3K15 | MLK7 | + | + | |||
MAP3K16 | TAOK1 | MAP2K3 | + | |||
MAP3K17 | TAOK2 | MAP2K3 | + | |||
MAP3K18 | TAOK3 | MAP2K3 | + | |||
MLK4 | + | + | ||||
Mos | + | |||||
aRaf | MAP2K1/2 | + | ||||
bRaf | MAP2K1/2 | + | ||||
cRaf | cRaf | MAP2K1/2 | + | + |
Kinases Mos and Raf (aRaf–cRaf) are activated by growth factors. Members of the RAF family are components of ERK signaling modules, as they phosphorylate MAP2K1 and MAP2K2. Kinases of the RAF family are recruited to the plasma membrane, where they links to small GTPase Ras and are phosphorylated (activated). Enzyme MAP3K8 also activates the ERK pathway, especially in the immune response.
The MEKK and MLK families can be activated by Rho GTPases and Ste20-like protein kinases (Sect. 5.1). Upon apoptotic cues, MAP3K1 (MEKK1) can bear proteolysis (activation) by caspases that cleave MAP3K1 and release its pro-apoptotic catalytic C-terminus. Although they are mainly involved in stress signaling, some members of the MAP3K and MLK families regulate the ERK pathways. Enzymes MAP3K1 and MAP3K11 (MLK3) target ERK1 and ERK2 as well as MAP3K2 and MAP3K3 targets ERK5.
Enzymes MAP3K5 (ASK1) and MAP3K7 (TAK1) are components of JNK and P38MAPK signaling pathways. Enzyme MAP3K5 participates in apoptosis caused by oxidative stress. It is activated by dissociation from a redox-sensitive inhibitor thioredoxin. Enzyme MAP3K7 is activated by cytokines and connects to tumor-necrosis factor-receptor associated factor TRAF6 via adaptor TAK1- and TRAF6-binding protein TAB2 (MAP3K7IP2; MAP3K7–MAP3K7IP2–TRAF6 complex) to activate transcription factor NFκB and JNK. TAK1(MAP3K7)-binding protein TAB2 promotes TRAF6 ubiquitination. Like MAP3K7, cRaf and MEKKs are able to activate NFκB (Sect. 10.9.1), independently of MAPK enzymes.
6.5.1 Family of Raf Kinases
The RAF family (aRaf–cRaf) of proto-oncogene products are cytoplasmic Ser/Thr kinases that operate in cell growth and tissue development. Each Raf has a distinct expression profile. Isotype cRaf, or Raf1, is widespread, whereas aRaf and bRaf are restricted to some tissues: aRaf is mainly expressed in urogenital and gastrointestinal tracts and bRaf in neural, hematopoietic, splenic, and testicular tissues. The B-RAF gene is the most frequently mutated oncogene. The common substrates for all 3 Raf kinases are MAP3K1 and MAP3K2, dual-specificity kinases that target extracellular signal-regulated protein kinases ERK1 and ERK2.
Members of the RAF family share 3 conserved regions (CR1–CR3). The CR1 domain contains the Ras-binding motif. Both CR1 and CR2 have a regulatory function that mediates binding to Ras and other regulators. The CR3 sequence in the C-terminus possesses the Ser/Thr kinase domain [547].
Activation of Raf kinase includes translocation to the plasma membrane, induction of a conformational change by Ras GTPase, and phosphorylation. The subcellular compartmentation and activity of Raf kinase is regulated by cognate kinase and phosphatase, as well as scaffold proteins and other interacting proteins (Sect. 7.7).18 The catalytic function of Raf results from a specific mode of dimerization of its kinase domain, the side-to-side dimerization [549]. Pseudokinase related to Raf, kinase suppressor of Ras (KSR), participates in the formation of the Raf side-to-side heterodimers and can trigger Raf activation.
The Ras–Raf–MA2PK–ERK module is activated by the guanine nucleotide-exchange factor SOS that stimulates Ras GTPase. Small Ras GTPase recruits Raf to the plasma membrane to phosphorylate MAP2K, which phosphorylates (activates) ERK enzyme. Kinases aRaf to cRaf have different phosphorylation sites, so that they can be independently regulated.
Signaling based on MAPK modules requires scaffold19 IQ motif-containing GTPase activating protein IQGAP1 to modulate the activation of bRaf by EGF [550].20
The Ras–Raf–PI3K signaling pathway leading to protein kinase-B can act either synergistically with or in opposition to the Ras–Raf–ERK pathway. PKB phosphorylates Raf, which then can inhibit activation of the Ras–Raf–ERK pathway. Cross-action between the Ras–Raf–ERK and Ras–Raf–PI3K–PKB pathways modulates cell life modes [551] (Sect. 5.2).
Some molecules can have 2 opposing mechanisms of action according to the cellular context and genotype, as they act as inhibitors or activators of Raf dimers [552]. Drug inhibitors that bind to Raf isoforms can provoke Raf dimerization or Ras–Raf interaction and membrane localization. Drug inhibitors that connect to one member of Raf homo- (cRaf–cRaf) or heterodimers (cRaf–bRaf) inhibits one subunit, but transactivate the drug-free protomer [553].
6.5.1.1 aRaf
V-Raf murine sarcoma 3611 viral oncogene homolog aRaf21 interacts with small GTPase rRas, MAP2K2, MAP2K-like protein PDZ-binding kinase (PBK), casein kinase-2β, phosphoinositide 3-kinase regulatory subunit PI3Kr1, as well as 14-3-3γ, 14-3-3η, and 14-3-3σ, among others [251]. Hence, whereas bRaf and cRaf bind to all the 14-3-3 isoforms, aRaf links to some isoforms (14-3-3ε, -σ, and -τ [547]) in vitro and to a lesser degree. Enzyme aRaf transduces signals from mitogens and growth factors and activates MAP2K1.
Like other RAF family members, aRaf binds lipids to enable membrane connection, thereby regulating the kinase activity. Like cRaf, aRaf tethers to monophosphorylated phosphoinositides (PI(3)P, PI(4)P, and PI(5)P) and phosphatidylinositol (3,5)-bisphosphate. In addition, aRaf also links specifically to PI(4,5)P2 and PI(3,4)P2 and to phosphatidic acid [547].
Like cRaf, 2 ARAF genes exist in the human genome, one of which is functional (ARAF1). The ARAF2 gene is a pseudogene [547]. Two alternative splice aRaf variants have been detected without kinase domain (but with the Ras-binding domain): daRaf1 and daRaf2. They bind to activated Ras, but act as antagonists of the Ras–ERK pathway.
Activity of aRaf is regulated by phosphorylation as well as lipidic and proteic interactions. Protein aRaf is able to form homo- and heterodimers as well as trimers with cRaf and bRaf. Heterodimer Raf has properties distinct from those of Raf monomer and homodimer, such as elevated kinase activity toward MAP2K.
Enzyme aRaf exhibits different effects according to the type of receptor Tyr kinase. It is recruited to EGFR upon EGF stimulation. However, it is constitutively associated with PDGFR.
Enzyme aRaf is weakly activated by small GTPase hRas and Src kinase with respect to bRaf and cRaf.22 Full aRaf activation needs Ras GTPase and other tyrosine kinases. Kinase aRaf may have the lowest kinase activity among Raf kinases for both MAP2K1 and MAP2K2 [547].
Casein kinase CK2β activates aRaf. Interleukin-3 and phosphoinositide 3-kinase contribute to aRaf activity. Kinase aRaf interacts with P85 regulatory subunit of PI3K (P85PI3K) in the presence or not of growth factor [547]. It also regulates the transition from the inactive dimeric form to the active tetrameric form of pyruvate kinase-M2. Enzyme aRaf interacts with TOM and TIM proteins involved in the mitochondrial transport system. It also targets trihydrophobin-1, a component of the negative elongation factor complex that hinders transcriptional elongation by RNA polymerase-2. In addition, aRaf acts as a dynamic interactor of scaffold Kinase suppressor of Ras KSR2 [547]. Protein aRaf binds constitutively to pro-apoptotic kinase mammalian Sterile 20-like kinase STK3 (MST2) [547].
Other interactors include [251, 547]: argininosuccinate synthase ASS1,23 Rab geranylgeranyltransferase β subunit, negative elongation factor proteins NElFc24 that precludes transcriptional elongation by RNA polymerase-2, translocase of inner mitochondrial membrane homolog TIMM44, PRP6 pre-mRNA processing factor PRPF6 (or U5 small nuclear ribonucleoprotein particle-binding protein) that is involved in pre-mRNA splicing, nucleoside uridine diphosphate-linked moiety X-type motif NUDT14, lymphokine-activated killer T-cell-originated protein kinase (TOPK or PBK), COP9 signalosome complex subunit-3, mitochondrial carbamoyl-phosphate synthase CPS1, and EGF-containing fibulin-like extracellular matrix protein EFEMP1 (or fibulin-3).
6.5.1.2 bRaf
V-Raf murine sarcoma viral oncogene homolog-B1 bRaf is also known as BRaf1, and RafB1. The Ras–Raf–ERK signal transduction pathway controls multiple processes, such as proliferation, differentiation, senescence, and apoptosis, depending on the duration and strength of the external stimulus and the cell type. The Ras–Raf–ERK pathway is activated in most human tumors, especially tumor cells with bRaf25 or Ras gene mutations. When the MAPK cascade is inhibited, tumor growth is completely hindered in bRaf mutant and only partly in Ras-mutant tumors [554]. In addition, bRaf, but neither aRaf nor cRaf, contributes to vascular patterning in the placenta, although Raf kinases play redundant roles during initial stages of embryogenesis [556].
Kinase bRaf interacts with kinases PKB, ERK1, and cRaf, small GTases hRas and mRas, Rap1 GTPase-activating protein, Ras-related protein RaP1A, and 14-3-3β, -γ, -ε, -ζ, -σ, and -τ [251]. It mobilizes ERK1 and ERK2 via MAP2K1 and MAP2K2 and potentially ERK3.
6.5.1.3 cRaf
Protein Ser/Thr kinase (MAP3K) cRaf, or Raf1 (v-Raf1 murine leukemia viral oncogene homolog), binds and operates downstream from membrane-associated Ras GTPase. Activated cRaf phosphorylates (activates) dual-specificity protein kinases MAP2K1 and MAP2K2 that, in turn, phosphorylate (activate) extracellular signal-regulated kinases ERK1 (MAPK3) and ERK2 (MAPK1 or MAPK2). The kinetics (sustained or transient activation) and intensity of Raf–ERK pathway stimulation by growth factors specify the signaling type. Kinase cRaf is a direct effector of Ras GTPase. Its activation involves plasmalemmal recruitment and binding to RasGTP. It is inactivated by protein phosphatase-5 (Sect. 8.3.5) [555].
Pleiotropic cRaf interacts with receptor PDGFRB, dihydrotestosterone receptor, nuclear receptor NR3c1, transcription factor Myc, G-protein subunits Gβ2 and Gγ4, monomeric GTPases Rap1a, eRas, hRas, kRas, mRas, nRas, rRas, RasD2, and RHEB, guanine nucleotide-exchange factor Vav1, arrestin-β2, kinases MAP3K5 (ASK1), MAP2K1, MAPK1 (ERK2), MAPK3 (ERK1), MAPK7 (ERK4), Mst4 (or MASK), bRaf, Src, Fyn, LCK, LTK, JaK1, JaK2, PAK1, PKG1, and PKCε, phosphatase CDC25A, phosphorylase kinase-α2, lipoprotein receptor-related protein associated protein LRPAP1, TNFR2–TRAF-signaling complex proteins Inhibitor of apoptosis proteins IAP2 and IAP3,26 adaptor GRB10, interactor MAPK8IP3 (or JIP3), connectors enhancers of kinase suppressor of Ras CnKSR1 and CnKSR1, phosphatidylethanolamine-binding protein PEBP4, sprouty-2, transcriptional modulator prohibitin, transcriptional regulator TSC22-related protein TSC22D3, Soc2 suppressor of clear homolog SHOC2, chaperonin-containing TCP1 subunit CCT3, heat shock protein Hsp90α, heat shock protein-A-binding protein-2, voltage-dependent anion channel VDAC1 (porin), ubiquitin-conjugating enzyme UbE2d2, ubiquitin ligase CHIP (or STUB1), and 14-3-3β, -γ, -ζ, -η, and -τ [251].
Kinase cRaf is a regulator of apoptosis, as it can phosphorylate (inactivate) pro-apoptotic protein BCL2 antagonist of cell death (BAD) in addition to potentiating BCL2-mediated resistance to apoptosis. It connects to retinoblastoma proteins Rb1 and Rb2, B-cell lymphoma protein BCL2, BCL2-associated athanogene BAg1 that enhances BCL2 anti-apoptotic effect, BCL2-like protein BCL2l1, and caspase-like apoptosis regulatory protein ClARP (Caspase homolog [CasH], Casp8 and FADD-like apoptosis regulator [CFLAR], or usurpin-β) [251].
6.5.2 Moloney Sarcoma Viral Mos Proto-Oncogene Kinase (Mos)
Kinase Mos is also a MAP3K specific to the ERK pathway, but it operates mainly in the reproductive system, as it is primarily expressed in germ line cells. It is expressed early during oocyte maturation and disappears immediately after fertilization. It actually contributes to oocyte maturation, as it activates ERK1 and ERK2 and maturation-promoting factor, a heterodimeric CDK1–cyclin-B complex that triggers oocyte maturation. As a cytostatic factor (CsF), it controls meiosis like maturation-promoting factor (MPF) for the cell division cycle.
6.5.3 Family of Mixed Lineage Kinases
The family of mixed lineage kinases (MLK) include MLK1 (MAP3K9), MLK2 (MAP3K10), MLK3 (MAP3K11), dual leucine zipper-bearing kinase (DLK or MAP3K12), LZK (MAP3K13), MLK4, MLK7α (MRKα, MLTKα, or ZAKα) and MLK7β (MRKβ, MLTKβ, or ZAKβ). These Ser/Thr kinases also belongs to Tyr kinase-like (TKL) superfamily of protein kinases. They activate stress-activated protein kinases Jun N-terminal kinases via MAP2K4 (JNKK1) and MAP2K7 and P38MAPKs via MAP2K3 and MAP2K6, as well as ERK1 and ERK2 via MAP2K1 and MAP2K2. Mixed lineage kinases also cause mitochondrial cytochrome-C release and caspase activation.
Members of the MLK family — MLK3 and MLK7 — are detected in adult hearts. In cardiomyocytes, MLK7 is activated in response to β-adrenergic stimulation and targets the P38MAPK and JNK axes. Saturated free fatty acid causes metabolic stress and activates JNK signaling via MLK3, MLK4, and MLK7 [556].
6.5.3.1 Mixed Lineage Kinase MLK1 – MAP3K9
Mitogen-activated protein kinase kinase kinase-9 (MAP3K9)27 contains an N-terminal glycine-rich region, an SH3 domain, a kinase domain similar to both Tyr and Ser/Thr kinases, 2 Leu/Iso (or Ile) zipper motifs, and a polybasic sequence similar to nuclear localization signals near the C-terminus [557, 558]. Kinase MLK1 requires autophosphorylation of its activation loop (Thr304, Thr305, Ser308, and Thr312) for full activity. It is predominantly produced in the central nervous system, kidney, spleen, stomach, and testis [559].
Kinase MLK1 phosphorylates dual-specificity kinase MAP2K4 to activate Jun N-terminal kinases in coordination with pro-apoptotic scaffold proteins such as Plenty of SH3 domains POSH1, especially for JNK-mediated neuronal apoptosis [559]. Scaffold POSH interacts with activated Rac to stimulate a subset of MLK family members, notably CDC42–Rac-interactive binding domain-containing MLK1 to MLK3. It forms a complex with MAP3K kinase (MLK1–MLK3 or MAP3K12), MAP2K (MAP2K4 or MAP2K7), and JNK1 or JNK2. It is inhibited by PKB. It operates as a pro-apoptotic protein in fibroblasts and mature neurons.
6.5.3.2 Mixed Lineage Kinase MLK2 – MAP3K10
Enzyme MAP3K1028 possesses a structure similar to that of MAP3K9, i.e., an SH3 sequence, a kinase motif that is associated with both Tyr and Ser/Thr specificity, a double Leu/Iso zipper region, and a basic domain similar to nuclear localization signals, as well as a large C-terminus. The production of MAP3K10 is relatively restricted, as it is predominantly detected in the central nervous system, kidney, skeletal muscle, and testis [560]. Highest expression level is observed in brain and skeletal muscles.
In neurons, huntingtin interacts with MAP3K9 and huntingtin-associated protein HAP1 that connect to neuron-specific basic helix–loop–helix transcription factor neurogenic differentiation protein NeuroD1 (or β-cell E-box transactivator β2).29 Enzyme MAP3K9 phosphorylates (activates) NeuroD [561]. This effect is facilitated by huntingtin and huntingtin-associated HAP1 protein.
Other MAP3K10 interactors include MAP2K4, MAPK8IP1 and MAPK8IP2, CDC42, dynamin-1, kinesin-3A, kinesin-associated protein KiFAP3, clathrin heavy chain-1, Disc large homolog DLg4, keratin-associated protein-3 pseudogene product KrtAP3P1, human growth factor-regulated tyrosine kinase substrate (HGS or HRS), hippocalcin, and 14-3-3ε [251].
Active small GTPases CDC42 and Rac1 tether MAP3K10 and might then relieve its auto-inhibition. P21 (CKI1a)-activated kinase PAK1 binds MAP3K10 to partly control its capacity to activate JNK [560]. The Rho target and scaffold Connector enhancer of kinase suppressor of Ras CnKSR1 connects to MAP3K10 possibly for Rho activation of JNK kinase. Ubiquitin conjugase UbE2d3.2 expressed in a tissue-restricted fashion during development interacts with MAP3K10 kinase [560].
Although it can phosphorylate MAP2K3 and MAP2K6, MAP3K10 kinase is a weak activator of the P38MAPK and ERK pathways [560]. Its major substrates include MAP2K4 and MAP2K7. It is rather specific to the JNK pathway, operating as a JN3K kinase. Members of scaffold POSH and JIP families that can dimerize bind directly to MAP3K10 to facilitate the activation of the JNK pathway. Conversely, JNK2 phosphorylates MAP3K10 kinase.
MAP3K10 participates in the regulation of vesicular transfer. It localizes to clathrin-coated vesicles [560]. It binds to dynamin-1. In addition, like MAP3K9, MAP3K10 is involved in neuronal apoptosis.
6.5.3.3 Mixed Lineage Kinase MLK3 – MAP3K11
Enzyme MAP3K1130 is widespread. It has a structure identical to MLK1 and MLK2 enzymes. Enzyme MAP3K11 colocalizes with prenylated, activated monomeric GTPase CDC42 at the plasma membrane [562]. Its activation requires phosphorylation of multiple sites in the activation loop.
Enzyme MAP3K11 activates the JNK pathway in response to gonadotropin-releasing hormone, epidermal and platelet-derived growth factors, tumor-necrosis factor-α, transforming growth factor-β, T-cell receptor costimulation, and interferon-γ [562]. It contributes to cell proliferation, especially to microtubule organization during the cell division cycle.
Enzyme MAP3K11 can be phosphorylated (activated; Ser281) by MAP4K1 (or hematopoietic progenitor kinase HPK1) [560]. In addition to MAP3K1, MAP3K11 is, indeed, another effector for MAP4K1 that also interacts with adaptors GRB2, CRK, and CRKL for signaling, as they recruit MAP4K1 to autophosphorylated receptor Tyr kinases at the plasma membrane. Enzyme MAP3K11 can also tether directly to CDC42 [267]. Enzyme MAP4K2 (GCK) interacts directly with and activates MAP3K11 kinase [560].
Substrates of MAP3K11 include MAP2K1, MAP2K3, as well as MAP2K4 and MAP2K7 that activate ERK, P38MAPK, and JNK, respectively [562]. Type of scaffold proteins directs MAP3K11 signaling mode. MAPK-interacting proteins, such as JNK-interacting proteins JIP1 and JIP3 as well as SH3 domain-containing ring finger protein SH3RF1,31 promote MAP3K11-mediated JNK activation, whereas JIP2 enhances MAP3K11-induced P38MAPK stimulation [562]. In addition, MAP3K11 phosphorylates IκB kinases IKKα and -β.
Substrates of PKB comprise glycogen synthase kinase-α and -β, cRaf, bRaf, MAP3K5, MAP3K11, and MAP2K4, as well as Fox transcription factors. In addition, PKB1 isoform (but not PKB2) interacts with scaffold JNK-interacting protein JIP1 and prevents formation of JNK signaling complex. On the other hand, PKB2 targets SH3RF1 scaffold for the JNK signaling axis. This scaffold binds to activated Rac and is able to activate the JNK pathway and promote nuclear translocation of NFκB factor. Protein kinase-B2 binds to the SH3RF1–MAP3K11–MAP2K–JNK complex and phosphorylates MLK3, thereby causing complex disassembly and impeding the JNK pathway [564].32
Small Rho GTPases, such as CDC42 and Rac, as well as MAP4Ks, such as MAP4K1 and MAP4K2, provoke MAP3K11 activity. Binding of a GTPase to the CDC42–Rac-interactive binding site (CRIB) disrupts auto-inhibition generated by the SH3 domain of MAP3K11 on its kinase motif. Ceramide also activates MAP3K11. Dimerization or oligomerization enhances MAP3K11 function. Conversely, MAP3K11 phosphorylation (Ser674) by PKB lowers its catalytic activity. Kinase-specific cochaperone Hsp90 regulates MAP3K11 stability.
6.5.3.4 Mixed Lineage Kinase DLK – MAP3K12
Enzyme MAP3K1233 activates the JNK (SAPK) pathway via MAP2K7 enzyme. It is involved in the regulation of apoptosis, cAMP response element-binding protein transcriptional activity, and terminal differentiation of keratinocytes [565]. It can form homodimers. Oligomerization-dependent autophosphorylation is required for its activation.
Unlike most of the MLKs that are widely expressed, MAP3K12 exhibits a more restricted pattern of expression. It activates JNK for neuronal migration during cerebral cortex development [556]. Scaffolds MAPK-binding inhibitory proteins MAPK8IP1 (or JNK-interacting protein JIP1) and MAP3K12IP1 (or MUK-binding inhibitory protein [MBIP]) regulate MAP3K12 activity by preventing its oligomerization and activation. Protein phosphatases PP1 and PP2A dephosphorylate MAP3K12.
6.5.3.5 Mixed Lineage Kinase LZK – MAP3K13
Leucine zipper-bearing kinase (LZK), or MAP3K13, activates the Jun N-terminal kinase pathway, but not the ERK axis. It is detected in the human brain.
Enzyme MAP3K13 interacts with itself and MAP2K7, as well as mitogen-activated protein kinase-8 interacting protein MAPK8IP1 (or JNK-interacting protein JIP1) and peroxiredoxin-3 [251]. Enzyme MAP3K13 stimulates inhibitor of nuclear factor-κB kinase IKKβ, hence transcription factor NFκB. Anti-oxidant protein AOP1 that localizes to mitochondria binds to MAP3K13 to modulate its activity. Protein AOP1 enhances MAP3K13-induced NFκB activation [566].
6.5.3.6 Mixed Lineage Kinase MLK4
Mixed lineage kinase-4 (MLK4) has several splice variants. Isoform MLK4α contains a different, shorter C-terminus than MLK4β subtype. Its subcellular compartment comprise the plasma membrane and cytoplasm.
6.5.3.7 Mixed Lineage Kinase MLK7
Mixed lineage kinase-7 (MLK7)34 is ubiquitous. In cells, it is located in the cytosol and nucleus [567]. Two splice variants exist: MLK7α and MLK7β.
In response to stress, both isoforms phosphorylate MAP2K4 and MAP2K7 to activate the JNK pathway as well as MAP2K3 and MAP2K6 to excite the P38MAPK axis [567]. Overexpression of MLK7α causes cell proliferation and transformation as well as degradation of stress fibers. In the heart, MLK7β induces cardiac hypertrophy and fibrosis. Enzyme MLK7α, but not MLK7β, phosphorylates histone-3 in response to epidermal growth factor cue. Enzyme MLK7β contributes to cell cycle arrest after DNA damage.
Enzyme MLK7α is phosphorylated by PAK1. Enzyme MLK7β phosphorylates checkpoint kinase ChK2 [567]. Osmotic shock and ionizing radiation cause MLK7β autophosphorylation (activation; Thr161 and Ser165).
6.5.4 Family of MAP/ERK Kinase Kinases (MEKK)
The main signaling pathways that are activated by MAP3K1 to MAP3K3 include ERK1 and ERK2, ERK5, JNK, and P38MAPK (Table 6.15). Enzymes MAP3K1, MAP3K2, and MAP3K3 participate in cell proliferation and differentiation, and tissue development. Enzyme MAP3K1 is particularly involved in epithelial cell migration; MAP3K2 in T-cell response; MAP3K3 in angio- and cardiogenesis [556].
MAP3K | MAP2K | MAPK | Effect |
---|---|---|---|
MAP3K1 | MAP2K1 | ERK1/2 | Cell proliferation and differentiation |
MAP2K4/7 | JNK | Cell migration and apoptosis inhibition | |
MAP3K2 | MAP2K5 | ERK5 | Cell survival and proliferation |
MAP2K4/7 | JNK | Cell migration and apoptosis inhibition | |
MAP3K3 | MAP2K1 | ERK1/2 | Cell proliferation and differentiation |
MAP2K5 | ERK5 | Cell survival and proliferation | |
MAP2K4/7 | JNK | Cell migration and apoptosis inhibition | |
MAP2K3/6 | P38MAPK | Cell differentiation and apoptosis inhibition |
Two MAP3K axes determine vascular patterning: (1) bRaf–MAP2K1–ERK2 cascade that regulates blood vessel formation in the placenta and (2) MAP3K3–MAP2K5–ERK5 axis that controls vessel development in the embryo. Several MAP3Ks, such as MAP3K1, MAP3K5 (ASK1), and MLK7, can be involved in mature heart homeostasis.
6.5.4.1 MAP3K1 (MEKK1)
Mitogen-activated protein kinase kinase kinase MAP3K1 is a ubiquitous Ser/Thr kinase that transduces many mitogenic and metabolic stimuli, such as insulin and growth factors, as well as pro-inflammatory cues. Enzyme MAP3K1 regulates diverse functions in a tissue- and cell type-specific manner. In most cases, MAP3K1 preferentially activates MAP2K4 and MAP2K7 that, in turn, stimulate Jun N-terminal kinases and/or P38MAPKs [568].35 It can also activate extracellular signal-regulated kinases ERK1 and ERK2 via MAP2K1 and MA2PK2 enzymes. Besides, it can phosphorylate IκB kinases.
Protein MAP3K1 not only phosphorylates (activates) its effector kinases, but also has a ubiquitin ligase activity for ERK1 and ERK2 kinases. It also acts as a scaffold protein that binds to components (e.g., cRaf, MAP2K1, and ERK2) of the 3-tier ERK module as well as to the module activator Ras. It also uses additional scaffold proteins such as MAPK8IP3 to regulate the signaling kinetics and threshold.
Numerous input signals trigger MAP3K1 activation, such as osmotic stress, growth factors, and TNFSF proteins. Context-specific signaling of MAP3K1-based modules requires specific scaffold proteins to yield signaling routes with milestones made of proteic complexes.
WD repeat domain-containing protein WDR6836 couples MAP3K1 to 2 protein Ser/Thr kinases: dual-specificity Tyr phosphorylation-regulated kinase DYRK1a and DYRK1b and homeodomain-interacting protein kinase HIPK2 [569].37 Proteins MAP3K1 and DYRK1a suppress NFAT signaling, as NFAT is sequestered into the cytoplasm, thereby counteracting T-cell activation. Both MAP2K1 and HIPK2 target P300 acetyltransferase.38 In addition, both MAP3K1 and HIPK2 can bind to axin adaptor.39
It is a key MAP3K in the regulation of cell migration, especially that involved in vascular remodeling as well as epithelial cell movement primed by developmental factors FGF2, TGFβ, and activin B.
Enzyme MAP3K1 contributes to heart hypertrophy triggered by Gq subunit that is activated by angiotensin-2, endothelin, and adrenergic signals. Furthermore, it protects cardiomyocytes from oxidative stress [556].
Enzyme MAP3K1 participates in the modulation of immune responses. It favors B-cell proliferation via TNFRSF5-dependent stimulation of JNK and P38MAPK.40 In T lymphocytes, MAP3K1 precludes T-cell-mediated autoimmune diseases.
Enzyme MAP3K1 is a prosurvival and anti-apoptotic factor in a cell-type- and stimulus-dependent manner, but when overexpressed, it can be pro-apoptotic [568]. In addition, MAP3K1 can phosphorylate transcription factors such as STAT3 and cofactors, such as transducer of regulated CREB activity TORC1 (but not TORC2) and P300. Enzyme MAP3K1 stimulates P300-dependent transcription; P300 mediates apoptosis.
Enzyme MAP3K1 interacts with MAP4K1 (HPK1), MAP4K2 (GCK), MAP4K4 (HGK or NIK), cRaf, MAP2K1, MAP2K4 (JNKK1), MAPK1 (ERK2), and MAPK8 (JNK1), as well as MAPK8IP3 [251]. Enzyme MAP3K1 is an effector for MAP4K1 that also interacts with adaptors GRB2, CRK, and CRKL for signaling, as they recruit MAP4K1 to autophosphorylated receptor Tyr kinases at the plasma membrane. Active GTPase CDC42GTP binds and promotes MAP3K1 homodimerization and activation [267]. Other interactors include nuclear factor-κB inhibitor kinase IKKβ, morphogen disheveled-2, Wnt inhibitor axin-1, adaptor GRB2, RhoGAP4, NCK-interacting kinase (NIK), focal adhesion kinase, actin-crosslinking protein α-actinin, TNFR-associated factors TRAF2 and TRAF6, FK506-binding protein FKBP5, ribosomal proteins RPL4, RPL30, and RPS13, cell division cycle protein CDC37, a chaperone that complexes with HSP90 and many kinases (CDK4, CDK6, Src, cRaf, MOK,41 and eIF2αK), heat shock protein HSP90α, ubiquitin conjugase UbE2l, and 14-3-3ε [251]. Various intracellular molecules function as activators of MAP3K1, such as RhoA GTPase, filamin-B, which organizes interferon signaling, TRAF2, and glycogen synthase kinase GSK3β, whereas protein Ser/Thr kinase STK38 and multifunctional Parkinson disease protein Park7 of the peptidase C56 family sequester MAP3K1 and hinder MAP3K1 activity [568]. Receptor-interacting protein Ser/Thr kinase RIPK1 phosphorylates MAP3K1 enzyme.
Enzyme MAP3K1 can also interact with small GTPases CDC42, Rac1, and Ras. Activation of MAP3K1 by protein Tyr kinases requires the coordinated recruitment of MAP3K1 and its adaptors and activators to the plasma membrane. Activated EGFR bound to phosphorylated adaptor SHC can recruit: (1) the GRB2–MAP3K1 complex; (2) adaptors CRK or CRKL and MAP4K1; or (3) the Ras-activating GRB2–SoS complex [267]. On the other hand, EPH receptor recruits and activates MAP4K4 and NCK.
6.5.4.2 MAP3K2 (MEKK2)
Enzyme MAP3K2 selectively activates JNK and ERK5 kinases. It also phosphorylates (activates) IκB kinases, thus initiating NFκB signaling. Enzyme MAP3K2 interacts with MAP2K4 (JNKK1), MAP2K5, MAP2K7 (JNKK2), and MAPK8 (JNK1), as well as T-lymphocyte specific adaptor SH2 domain-containing protein SH2D2a, apoptosis inhibitor IAP3,42 and 14-3-3ε and 14-3-3σ [251].
Enzyme MAP3K2 contributes to the maturation and function of cellular immunity, as it modulates T-cell receptor stimulation of the JNK pathway and favors cytokine production in response to IgE [556].
6.5.4.3 MAP3K3 (MEKK3)
Ubiquitous MAP3K3 regulates ERK1 and ERK2, ERK5, JNK, and P38MAPK signaling as well as NFκB transcription factor. It intervenes in cardiovascular development via MAP2K5 and ERK5, especially in the maturation of primary vasculature and endocardium. In addition, activation of ERK1 and ERK2, JNK, P38MAPK, and NFκB signaling pathways is necessary for epithelial-to-mesenchymal transition, particularly during the formation of the cardiovascular and nervous system [556].
Enzyme MAP3K3 interacts with MAP2K5 as well as microtubule affinity-regulating kinase-2 (MARK2). Other interactors include GRB2-associated binding protein GAB1, transcription factor zinc finger and BTB domain-containing ZBTB16, TNFR-associated factor TRAF7, P53- and DNA-damage-regulated protein PDRG1, cell division cycle homolog CDC37, DNA-damage repair mediator breast cancer susceptibility protein BrCa1, JNK-associated scaffold protein sperm-associated antigen SpAg9, prefoldin-2, heat shock proteins HSP90α (HSPCal3) and HSPa4, and 14-3-3γ and 14-3-3ε [251].
Hypertonicity (e.g., high NaCl concentration)43 activates the osmolarity-responsive NFAT5 transcription factor.44 Small Rac1 GTPase indeed complexes with cerebral cavernous malformation-2 homolog (CCM2)45 MAP3K3, and MAP2K3 that activates P38MAPK in response to hypertonicity. However, NFAT5 activation results from signaling via phospholipase-Cγ1 and the Rac1-CCM2 axis, but not the Rac1-CCM2-MAP3K3-MAP2K3-P38MAPK pathway [571].
6.5.4.4 MAP3K4 (MEKK4)
Enzyme MAP3K446 activates JNKs and P38MAPKs, but not MAP2K1, in response to environmental stresses, such as osmotic shock, UV irradiation, wound stress, and inflammatory factors. It is a major activator of the P38MAPK pathway, but a minor effector of the JNK pathway. The JNK signalosome is a complex constituted by Jun N-terminal kinase and dual-specificity kinases MAP2K4 and MAP2K7 that can activate Jun transcription factor, a AP1 component.
Enzyme MAP3K4 contributes to the regulation of cell proliferation, apoptosis, and migration. It participates in neural, cardiac (valvulogenesis, chamber partitioning, and formation of the coronary vasculature), and skeletal development [556]. Wnt signaling can operate via MAP3K4. Angiotensin-2 provokes calcium-dependent phosphorylation by FAK2 of MAP3K4 that, in turn, activates MAP2K6 in smooth muscle cells. In addition, both MAP2K6 and calcium-regulated phosphatase calcineurin (PP2B or PP3) are involved in T-cell signaling and activation.
Enzyme MAP3K4 interacts with MAP2K1, MAP2K3, MAP2K4 (JNKK1), and MAP2K6, small GTPase CDC42 and Rac1, and growth arrest and DNA-damage-inducible GADD45α, -β, and -γ [251]. These 3 GADD45 polypeptides may contribute to activation of both P38MAPKs and JNKs via MAP3K4 enzyme. However, GADD45 homologs remains at low level when JNKs are fully activated during DNA damage [267].
6.5.5 Family of Mitogen-Activated Protein Kinase Kinase Kinases-5/6
Mitogen-activated protein kinase kinase kinases MAP3K5 and MAP3K6 are also tagged apoptosis signal-regulating kinases ASK1 and ASK2 as well as MAP/ERK kinase kinase MEKK5 and MEKK6, respectively. They indeed regulate apoptosis downstream from cellular stresses.
6.5.5.1 MAP3K5 or ASK1
Enzyme MAP3K5, or apoptosis signal-regulating kinase ASK1, functions in both the MAP2K4–JNK (or JN2K1–JNK) and MAP2K7–JNK (or JN2K2–JNK), as well as MAP2K3–P38MAPK (or SKK2–P38MAPK) and MAP2K6–P38MAPK (or SKK3–P38MAPK) cascades. It intervenes in apoptosis under various stress types, such as oxidative and endoplasmic reticulum stresses. These stresses causes MAP3K5 homo-oligomerization and autophosphorylation (Thr838). Apoptosis that depends on MAP3K5 is mainly mediated by mitochondria-dependent caspase activation (caspase-9 and -3) [572].
Enzyme MAP3K5 also mediates signaling for cell differentiation and survival, in particular, in neurons and keratinocytes [572]. It is also involved in inflammation and innate immunity. Production of inflammatory cytokines (e.g., TNFα, IL6, and IL1β) relies on MAP3K5 in splenocytes and bone marrow-derived dendritic cells.
Oligomerization of MAP3K5 is required for its activation by trans-autophosphorylation. Enzyme MAP3K5 is phosphorylated (activated; Thr838) [572]. In addition, MAP3K6 hetero-oligomerizes with MAP3K5 (ASK1–ASK2 complex) and directly phosphorylates MAP3K5 (Thr838). The MAP3K5–MAP3K6 heteromer stabilizes MAP3K6 and improves MAP3K6 efficiency for its subtrates in the JNK and P38MAPK modules. The MAP3K5–MAP3K6 complex causes apoptosis.
Enzyme MAP3K5 interacts not only with itself and MAP3K6, but also with MAP3K7 (TAK1). It then disrupts TRAF6–MAP3K7 interaction and inhibits IL1-induced NFκB activity [572]. It connects to MAP2K6 and MAP2K7 [251]. It is also linked to MAPK8IP3 (or JNK-interacting protein JIP3), thioredoxin, glutaredoxin, TNFR-associated factors TRAF1 to TRAF3, TRAF5 and TRAF6, arrestin-β2, glutaminyl-tRNA synthase, glutathione S-transferase-M1, dual-specificity phosphatase DuSP19, cell division cycle CDC25a phosphatase, cRaf, growth arrest and DNA-damage-inducible protein GADD45β, cyclin-dependent kinase inhibitor CKI1a, programmed cell death PCD6,47 endoplasmic reticulum-to-nucleus signaling ERN1, and 14-3-3ζ protein, among others (Table 6.11).
Various factors regulate MAP3K5 oligomerization. Both TRAF2 and TRAF6 as well as adaptor death-associated protein DAP6,48 facilitate MAP3K5 oligomerization and activation. On the other hand, Vacuolar protein sorting-associated protein-28 homolog (VPS28)49 is an anti-apoptotic protein that suppresses MAP3K5 oligomerization [572].
Conversely, MAP3K5 is inactivated by phosphorylation at Ser83, Ser966, and Ser1033 in humans [572]. Protein kinase-B phosphorylates (inactivates) MAP3K5 (Ser83). Phosphoinositide-dependent protein kinase PDK1 also phosphorylates (inhibits) MAP3K5 (Ser966). The receptor Tyr kinase insulin-like growth factor receptor also phosphorylates (inactivates) MAP3K5. In addition, 14-3-3 protein prevents MAP3K5 activity, as it binds to a phosphorylated Ser residue (Ser966) in the 14-3-3-binding motif of MAP3K5 agent [572].
On the other hand, protein kinase-D and Disabled homolog 2-interacting protein (Dab2IP)50 foster the release of 14-3-3 inhibitor from MAP3K5 enzyme. Homeodomain-interacting protein kinase HIPK1 is an MAP3K5–Dab2IP complex-associated protein that activates MAP3K5, as it dissociates the inhibitors 14-3-3 and thioredoxin from MAP3K5. Kinase HIPK1 assists activation of the MAP3K5–JNK axis by TNFα and ROS agents [459].
In addition, Ca
–calmodulin-dependent protein kinase CamK2 phosphorylates (activates) MAP3K5 in neurons [573]. Ste20-like kinase (SLK) interacts with and activates MAP3K5 and provokes apoptosis [572].

Phosphatase PP2 supports MAP3K5 activity, as it dephosphorylates an inactivating phosphorylation site (Ser966) and dissociates 14-3-3 from MAP3K5 enzyme [572]. Calcium–calmodulin-activated protein phosphatase PP3 also activates MAP3K5, as it promotes its release from 14-3-3 proteins in cardiomyocytes. Moreover, PP3 dephosphorylates the inactivating phosphorylation site of MAP3K5, thereby exciting its activity. On the other hand, protein Ser/Thr phosphatase PP5 inhibits MAP3K5, as it dephosphorylates an activating phosphorylation site (negative feedback).
Phosphatase CDC25a inhibits MAP3K5 independently of its phosphatase activity, as it impedes MAP3K5 oligomerization [572]. Dual-specificity phosphatase DuSP1951 enhances MAP3K5 signaling, as it acts as a scaffold for this kinase.
Activation of the MAP3K5–P38MAPK pathway upon stimulation by reactive oxygen species is required for extracellular ATP-induced apoptosis in macrophages, which is initiated by the ATP-ligated P2X7 receptor, an ATP-gated ionotropic channel [572].
Adaptors TRAF2 and TRAF6, which link plasmalemmal receptors and kinases, are recruited to the ROS-stimulated MAP3K5 signalosome and activate MAP3K5 (full MAP3K5 activation), after dissociation of thioredoxin from inactivated MAP3K5, the MAP3K5 signalosome enlarging with respect to its resting state [572].
Redox sensing oxidoreductase thioredoxin, only in its reduced state, inhibits MAP3K5, as it sequesters MAP3K5 away from TRAF2 until TRAF2-dependent production of reactive oxygen species provokes the conversion of thioredoxin to its oxidative form, hence the dissociation of the MAP3K5–thioredoxin complex [267]. Interaction between MAP3K5 and TRAF6 is also prevented by thioredoxin.
Calcium- and integrin-binding protein-1 (CIB1)52 that interacts with α2B integrin in particular, binds to MAP3K5, interferes with the recruitment of TRAF2 to MAP3K5, and inhibits MAP3K5 autophosphorylation, thereby repressing MAP3K5 activation [573]. Inhibitor CIB1 thus competes with TRAF2 to connect to MAP3K5 kinase. Calcium influx relieves inhibition of CIB1 on MAP3K5 kinase.
Retinoblastoma RB1-inducible coiled-coil protein RB1CC1,53 a widespread inhibitor of FAK1 and FAK2 and regulator of the retinoblastoma gene54 contributes to the regulation of cell proliferation and migration as well as regulates TRAF2–MAP3K5 interaction [575].55 Eukaryotic translation initiation factor-2α kinase-2,56 an element of the antiviral response, links to MAP3K5 to activate the JNK and P38MAPK pathways [576].
Enzyme MAP3K5 is also regulated by ubiquitination. Suppressor of cytokine signaling SOCS1 constitutively binds to MAP3K5 and causes its ubiquitination and degradation in resting cells [576]. Cellular inhibitor of apoptosis protein IAP157 is a ubiquitin–protein ligase for MAP3K5 that can terminate TNFα-induced MAPK activation (negative feedback).
Balance between cell survival and death relies on activation of the PI3K–PKB and MAP3K5–JNK pathways. Activated MAP3K5 triggers cell death in response to diverse apoptotic stimuli, such as TNFα or reactive oxygen species. Scaffold protein Disabled homolog Dab2-interacting protein (Dab2IP), a Ras-GTPase activating protein (RasGAP)58 causes cell cycle arrest and promotes apoptosis in response to stress. It actually suppresses the PI3K–PKB pathway and enhances MAP3K5 activation to impede cell survival and provoke cell death [577].59
Enzyme MAP3K5 interacts with cell cycle regulators. Cyclin-dependent kinase inhibitor CKI1a links to MAP3K5 and inhibits the MAP3K5 signaling [572].
Several scaffold proteins, such as MAPK8IP3 and β-arrestin, interact with MAP3K5 to facilitate its signaling [572]. The scaffold Gem (nuclear Gemini of Cajal bodies)-associated protein Gemin-5, a component of the survival of motor neurons (SMN) complex,60 interacts with ASK1 and potentiates activation of the MAP3K5–MAP2K4–JNK1 pathway. Parkinson disease protein Park7 tethers to and inhibits MAP3K5 enzyme.
Enzyme MAP3K5 phosphorylates Programmed cell death PDCD6,61 a member of the pentaEF hand Ca
-binding protein family; this phosphorylation is counteracted by cRaf [572].

Enzyme MAP3K5 contributes to cardiogenesis, as cardiac chamber partitioning and valve formation require appropriate timing of apoptosis, as well as left ventricular remodeling by inducing apoptosis after myocardial infarct via the P38MAPK and JNK pathways [556].
6.5.5.2 MAP3K6 or ASK2
Enzyme MAP3K6 interacts with MAP3K5 (ASK1). Alone, MAP3K6 weakly activates JNKs and does not stimulate ERKs and P38MAPKs [578]. Its kinase activity happens only in association with MAP3K5 enzyme. The latter may confer kinase activity to MAP3K6; it may allosterically activate MAP3K6, hence causing its autophosphorylation (Thr806). Activation of MAP3K6 does not depend on MAP3K5 kinase activity. In the presence of MAP2K5, MAP3K6 phosphorylates (activates) MAP2K4 and MAP2K6, thereby priming the MAP2K4–JNK and MAP2K6–P38MAPK pathways, to the same extent as MAP3K5 alone [578]. Reactive oxygen species leads to MAP3K6 autophosphorylation (Thr806) [578].
In addition to its heteromerization with MAP3K5, MAP3K6 can homodimerize. This homodimer may enable MAP3K6 trans-autophosphorylation. Protein 14-3-3 is a MAP3K6-interacting partner; this connection is reversibly regulated by phosphorylation.
Enzyme MAP3K6 regulates the expression of vascular endothelial growth factor under both normoxia and hypoxia in vitro [579]. Consequently, MAP3K6 favors tumor angiogenesis and tissue response to ischemia.
6.5.6 Mitogen-Activated Protein Kinase Kinase Kinase-7 (TAK1)
Mitogen-activated protein kinase kinase kinase-7 (MAP3K7) is also called transforming growth factor-β-activated kinase TAK1. This signaling mediator of innate immunity is activated by pro-inflammatory cytokines, such as interleukin-1 and tumor-necrosis factor-α, as well as transforming growth factor-β, bone morphogenetic protein BMP4, and Wnt family ligands [580]. Enzyme MAP3K7 contributes to vasculature development. It is also involved in the redox regulation.
Ubiquitous MAP3K7 is mainly located in the cytoplasm. Ligand Wnt1 causes MAP3K7 accumulation in the nucleus. Proteasomal degradation provokes a negative feedback. In humans, 4 splicing variants exist (MAP3K7a–MAP3K7d). Isoform MAP3K7a (i.e., MAP3K7) is the predominantly expressed form.
In mammalian cells, MAP3K7 activates NEMo-like kinase and downregulates transcriptional activation by β-catenin and T-cell factor/lymphoid enhancer factor [580]. Enzyme MAP3K7 interacts with upstream kinases MAP4K1 and MAP4K4 on the one hand and downstream enzymes MAP2K6 on the other [251]. It also connects to components of the same tier of MAPK module MAP3K5 and MAP3K14. In addition, MAP3K7 links to IκB kinases IKKα and IKKβ.
Enzyme MAP3K7 is required for the activation of JNK and nuclear factor-κB upon signaling from IL1-, TNF-, and Toll-like receptor. Upon IL1 signaling, MAP3K7 is recruited to TRAF6 complex. Mediator TRAF6 bears Lys63-linked ubiquitination that facilitates MAP3K7 activation. Activated MAP3K7 then stimulates the JNK and P38MAPK axes as well as IκB kinases to activate nuclear factor-κB [580].
Proteins MAP3K7 and TRAF6 also attract receptor activator of nuclear factor-κB, or tumor-necrosis factor-related activation-induced cytokine (TRANCE), that is expressed on activated T cells to stimulate mature dendritic cells as well as osteoblasts to prime osteoclastogenesis and osteoclast activation [580].
Two major MAP3K7-binding partners correspond to MAP3K7-interacting proteins MAP3K7IP1 and -2, or TAK1-binding proteins TAB1 and TAB2. Protein MAP3K7IP1 is an activator of MAP3K7, whereas MAP3K7IP2 is an adaptor that links MAP3K7 to TRAF6 [580]. Proteins MAP3K7IP1 and -2 participate in TRAF6-induced IKK activation. Enzyme MAP3K7 is constitutively associated with its activator MAP3K7IP1, but remains inactive in the absence of stimuli. Once stimulated by IL1, MAP3K7 autophosphorylates (activates; Thr184, Thr187, and Ser192).
Basal activity of MAP3K7 relies on autophosphorylation and its MAP3K7IP1 partner [581]. On the other hand, stimulus-dependent activity of MAP3K7 needs MAP3K7IP2. In particular, MAP3K7IP2 mediates polyubiquitin chain-dependent MAP3K7 activation in innate immunity. Autophosphorylation of MAP3K7 results from conformational changes following MAP3K7 association with MAP3K7IP2-polyubiquitin chains or MAP3K7IP1-dependent oligomerization. On the other hand, MAP3K7 is dephosphorylated by protein phosphatases PP2, PP6, and PPM1.
Inhibitor of apoptosis protein IAP3 binds MAP3K7IP1 and receptor Ser/Thr kinases BMPR1a (ALK3 or CD292) and BMPR1b (ALK6) that are targeted by BMP2, BMP4, BMP6, and BMP7 bone morphogenetic proteins, as well as GDF6 growth differentiation factor. It can then form a trimeric IAP3–MAP3K7IP1–MAP3K7 complex. Other members of the family of inhibitors of apoptosis can be recruited to TNFRSF complexes. Apoptosis inhibitors IAP1 and IAP2 interact with TRADD and TNFRSf1a (TNFR1) and can recruit MAP3K7.
Tumor-necrosis factor receptor-associated factor TRAF2 mediates TNF signaling, as it acts as an adaptor that relays signals in the NFκB and AP1 pathways. Upon TNF stimulation, TRAF2 is phosphorylated by PKC and, then, recruits IKKα and IKKβ to TNFR receptor. Phosphorylated TRAF2 also undergoes polyubiquitination that allows its connection to TAB2 and -3 (MAP3K7IP2 and -3) and activation of IKK and JNK [582].
Interleukin-17 receptor-D (IL17Rd)62 has both inhibitory and stimulatory activities. On the one hand, this transmembrane protein is a feedback inhibitor of fibroblast growth factor signaling [583].63 It can indeed interact with FGFR1 and prevent its phosphorylation as well as suppress Ras function, hence blocking FGF-mediated ERK activation. Its splice variant that gives rise to a cytoplasmic form of Sef likewise associates with FGFR1 and inhibits ERK activation. In addition, IL17Rd inhibits PKB. On the other hand, IL17Rd interacts with MAP3K7 to activate a MAP3K7–MAP2K4–JNK pathway [584].
Other MAP3K7 interactors include TGFβ receptor TβR1 (or activin receptor-like kinase ALK5), inhibitory SMAD6 and SMAD7, TNFRSF11a, magnesium-dependent protein phosphatase-1B, receptor (TNFRSF)-interacting Ser/Thr kinase RIPK1, cRaf kinase inhibitor protein (RKIP or phosphatidylethanolamine-binding protein PEBP1), hepatocyte growth factor-regulated tyrosine kinase substrate (HRS or HGS), myeloid differentiation primary response gene product MyD88 and Toll–IL1 receptor domain-containing adaptor protein (TIRAP), as well as cylindromatosis (Cyld) deubiquitinase [251].
Dysregulation in cellular redox systems (i.e., anti-oxidant enzymes, such as superoxide dismutase and catalase, and scavengers such as glutathione) causes accumulation of reactive oxygen species. Among molecules involved in redox regulation, transcription factors NFκB, AP1, and NRF2 regulate production of anti-oxidant enzymes. Enzyme MAP3K7 activates NFκB and AP1, especially after stimulation by cytokines and Toll-like receptors, and upregulates NRF2 [581].
6.5.7 Family of Thousand and One Kinases (TAOK)
Thousand and one kinases (TAOK1–TAOK3 or MAP3K16–MAP3K18; Sect. 5.1.10) that constitute the GCK subfamily 8 primarily activate the P38MAPK pathway in response to cellular stress and DNA damage.
Upon DNA damage, ataxia telangiectasia mutated kinase (ATMK) is activated and phosphorylates TAOK kinase. Phosphorylated TAOKs then activate P38MAPK and cells enter the G2–M damage checkpoint for DNA repair [556].
Kinase MAP3K16 activates MAP2K3 that targets P38MAPKs. It also targets JNKs. Kinase MAP3K17 prevents activation by MAP3K7 of the NFκB pathway after osmotic stress. It also associates with MAP2K3 and MAP2K6 that both targets P38MAPKs. It can also activate JNK kinases.
6.5.8 Mitogen-Activated Protein Kinase Kinase Kinase-8 (TPL2)
Mitogen-activated protein kinase kinase kinase-8 (MAP3K8)64 activates the ERK and JNK pathways in immunocytes stimulated by lipopolysaccharides and other inflammatory mediators. However, development of immunocytes (T and B lymphocytes, dendritic cells, natural killer cells, and macrophages) does not need MAP3K8 protein. Enzyme MAP3K8 is required for activation of ERK1 and ERK2 in myeloid cells after TLR (TLR2 and TLR9) and TNFRSF receptor stimulation. In resting cells, MAP3K8 complexes with inhibitory protein NFκB1 (P105NFκB monomer), thereby being unabled to connect to its MAP2K substrate. In stimulated cells, the IKK complex phosphorylates NFκB1, thereby triggering its ubiquitination by SCF ligase for proteasomal degradation and releasing MAP3K8 as well as Rel subunits for nuclear translocation [585]. During inflammation, MAP3K8 enables the production of pro-inflammatory cytokine TNFα. Enzyme MAP3K8 can also activate nuclear factor of activated T cells and prime interleukin-2 production.
The Tpl2 gene encodes full-length MAP3K8FL 65 and MAP3K8S variant66 by alternative translational initiation at methionine 1 (M1) or 30 (M30). C-terminal truncation increases protein stability and kinase activity; moreover, MAP3K8S is expressed at higher levels [585].
Transcript Tpl2 mRNA is expressed at highest levels in the lung, spleen, and thymus, and at low levels in the brain, liver and testis [585]. As Tpl2 mRNA is also detected in the kidney, salivary glands, stomach, intestine, and skeletal muscles, MAP3K8 is a widespread molecule. Expression of MAP3K8 is greater in the ventricular myocardium during body’s development than in adults [556].
The signaling function of MAP3K8 is regulated by phosphorylation. Autophosphorylation targets Thr290 residue. Additional phosphorylation (Ser400) is needed to activate MAP2K effector [585].
Enzyme MAP3K8 interacts with MAP3K14 as well as MAP2K1 and MAP2K4 to MAP2K6 effectors [251, 585]. It also links to IκB kinases IKKα67 and IKKβ, as well as nuclear factor-κB subunits (NFκB1, NFκB2, Rel, and RelA) [251]. Other MAP3K7 interactors comprise protein kinase-B, kinase suppressor of Ras KSR2, TNFR-associated factor TRAF2, and ubiquitin-binding TNFα-induced protein-3-interacting protein TNIP2.68 Enzyme MAP3K8 can be confined to a cytoplasmic complex with P105NFκB 69 and ubiquitin-binding TNFαIP3 protein.70
6.5.9 Mitogen-Activated Protein Kinase Kinase Kinase MAP3K14
Mitogen-activated protein kinase kinase kinase-14 (MAP3K14) is a TRAF2-interacting Ser/Thr kinase that shares sequence similarity with several other MAP3Ks and stimulates nuclear factor-κB. It actually participates in NFκB-mediated signaling triggered by receptors of the TNFRSF–NGFR set (Vol. 3 – Chap. 11. Receptors of the Immune System)71 family that activates or inhibits cell death and interleukin-1 type-1 receptor. It is thus branded nuclear factor-κB-inducing kinase (NIK).72 Besides, MAP3K14 seems to be exclusively involved in the activation of NFκB. Other MAP3K14 aliases comprise HS and HSNIK.
Upon stimulation from TNFRSF11a, TNFR1 and TNFR2, Toll-like receptors, T-cell receptors, CD3 and CD28 coreceptors, interleukin-1 receptor, and lipopolysaccharides, MAP3K14 activates IκB kinase, as it phosphorylates both NFκB inhibitors IKKα and IKKβ. Activated IKK then phosphorylates IκBα (Ser32 and Ser36) that leads to IκBα ubiquitination and degradation, thereby allowing NFκB translocation to the nucleus.
Enzyme MAP3K14 interacts not only with TRAF2, but also with TRAF1, TRAF3, and TRAF6 agents. It also connects not only to α and β subunits of inhibitor of nuclear factor-κB kinase, but also inhibitor of κlight polypeptide gene enhancer in B cells, kinase complex-associated protein (IKBKAP), and kinase-γ (IKBKγ), as well as nuclear factor-κlight polypeptide gene enhancer in B-cell inhibitor-α (NFKBIα). In addition, MAP3K14 associates with itself, MAP3K7 (or TAK1), and MAP3K8 (TPL2), as well as epidermal growth factor receptors (heregulins), TNFR-interacting protein kinase RIPK1, phosphatidylethanolamine-binding protein PeBP1 (or Raf1 kinase inhibitor protein [RKIP]), caspase-8 and -10, and caspase-8 and FADD-like apoptosis regulator (CFLAR) [251].
6.6 Mitogen-Activated Protein Kinase Kinases
Activated MAP2K phosphorylates a specific MAPK (Table 6.16). Activated MAPK phosphorylates cytosolic and nuclear effectors. MAPK members have, indeed, many substrates: (1) other protein kinases belonging to either downstream components ( MAPK-activating protein kinases MAPKAPK1 and MAPKAPK2, ribosomal S6-kinase (RSK1–RSK3) that phosphorylates glycogen synthase kinase GSK3) or upstream elements ( cRaf and SOS); (2) enzymes (phospholipases PLA2 and PLCγ); (3) plasmalemmal proteins, such as growth factor receptors (EGFR); and (4) transcription regulators, such as the serum response factor, Fos, Jun, MyC, and other transcription factors ( ATF2, ELk1, and MEF2), as well as members of the steroid/thyroid hormone receptors. Enzymes of the MAPK modules are inhibited by MAPK phosphatases (tyrosine phosphatases and serine/threonine phosphatases).
Table 6.16
Types of MAPK enzymes targeted by MAP2K proteins (MEK:mitogen–extracellular signal-regulated kinase; MKK: MAP kinase kinase). Activators of P38MAPKs comprise MAP2K3, MAP2K4, and MAP2K6, but MAP2K3 and MAP2K6 are the specific kinases of P38MAPK. MAP2K6 phosphorylates all P38MAPK isoforms (P38MAPKα–P38MAPKδ), whereas MAP2K3 preferentially activates P38MAPKα, P38MAPKγ, and P38MAPKδ (MEK: mitogen–extracellular signal-regulated kinase kinase; MKK: MAP kinase kinase; SEK: SAPK–ERK kinase SEK; SKK: SAPK kinase [SAPK: stress-activated protein kinase]).
MAP3K | Aliases | ERK1/2 | ERK5 | JNK | P38MAPK |
---|---|---|---|---|---|
MAP2K1 | MEK1, MKK1 | + | |||
MAP2K2 | MEK2, MKK2 | + | |||
MAP2K3 | MEK3, MKK3, SKK2 | + | |||
MAP2K4 | MEK4, MKK4, SKK1, | + | + | ||
JNKK1, SEK1 | |||||
MAP2K5 | MEK5, MKK5 | + | |||
MAP2K6 | MEK6, MKK6, SKK3 | + | |||
MAP2K7 | MEK7, MKK7, SKK4, | + | |||
JNKK2 |
6.6.1 MAP2K1
Enzyme MAP2K173 can be activated by MAP3K1 to MAP3K4, MAP3K8, and cRaf enzymes. It also interacts with itself as well as MAPK1 (ERK2) and MAPK3 (ERK1), kinase suppressor of Ras KSR2, MAP2K-interacting protein-1 and -3, mitogen-activated protein-binding protein-interacting protein (MAPBPIP), adaptor GRB10, phosphatidyl ethanolamine-binding protein PBP (or Raf kinase inhibitory protein) and PEBP4 (or cousin of RKIP1 protein CORK1), small GTPase hRas, guanine nucleotide-exchange factor Vav1, actin-binding protein filamin-α, and urokinase-type plasminogen activator (urokinase) [251].
6.6.2 MAP2K2
6.6.3 MAP2K3
Enzyme MAP2K375 is highly selective for P38MAPKs, but preferentially activates P38MAPKα (MAPK14), P38MAPKγ, and P38MAPKδ [587]. It is more heavily activated by physical and chemical stresses. Phosphorylation sites of MAP2K3 are Ser189 and Thr193 [267]. Enzymes MAP3K1, MAP3K3 to MAP3K5, MAP3K7, MAP3K11, as well as MAP3K16 to MAP3K18 (TAOK1–TAOK3) can activate MAP2K3. Stimulated MAP2K3 phosphorylates (activates) P38MAPK.
Enzyme MAP2K3 also interacts with MAP2K6, MAPK3 (ERK1), and MAPK-interacting protein MAPK8IP2, as well as phospholipase-Cβ2, transcription inhibitor SMAD7, and nephrin76 of the slit diaphragm of fenestrated endothelium of renal filtration barrier [251]. Interactor MAPK8IP2 that binds to RacGEFs TIAM1 and RasGRF1 activates Rac GTPase that can then stimulate MAP3K11 and MAP2K3 and P38MAPK kinases [587].
Enzymes MAP2K3 and P38MAPK associate with hepatocyte growth factor receptor (HGFR) and its partner Src kinase that stimulates Rac1 GTPase [587]. In addition, phosphorylated MAP2K3 activates minibrain-related kinase (MiRK or dual-specificity Tyr phosphorylation-regulated kinase DYRK1b) that phosphorylates (activates) the transcriptional regulator hepatocyte nuclear factor HNF1α, hence promoting cell proliferation. Conversely, P38MAPKα and P38MAPKβ bind to and disrupt the DYRK1b–MAP2K3 complex (negative feedback).
Activation of MAP2K3 is related to the stress-mediated remodeling of cytoskeletal proteins [587]. Osmosensing scaffold binds to actin, Rac GTPase, and MAP3K3 and MAP2K3 enzymes. Stress-mediated activation of MAP3K3 triggers the expression of several cytokines, such as interleukins IL1α, IL1β, IL6, and IL12 [587]. Lipopolysaccharides trigger the formation of a complex between IRAK2, TRAF6, MAPKAPK2, and MAP2K3 and MAP2K6 enzymes.
Activated MAP2K3 contributes to apoptosis [587]. Inhibitor SMAD7 interacts with the MAP3K7 (TAK1)–MAP2K3–P38MAPK complex in TGFβ1-induced apoptosis. Conversely, phosphorylated MAP2K3 can associate with anti-apoptotic factors immunoglobulin-binding protein IgBP1 and PP2 phosphatase. The latter dephosphorylates MAP2K3 and can suppress apoptosis [587].
Activated MAP2K3 can provoke clathrin-mediated endocytosis [587]. Platelet-activating factor induces the endocytosis of its receptor PAFR via the activation of the MAP2K3–P38MAPK cascade. Activation of PAFR actually triggers the formation of the PAFR–β-arrestin-1–clathrin complex, the simultaneous association of MAP2K3 with β-arrestin-1, and activation of the MAP2K3–P38MAPK signalosome.
6.6.4 MAP2K4
Enzyme MAP2K477 with MAP2K7 are crucial transducers that prime JNK signaling. It phosphorylates specific Tyr and Thr residues in the activation loop of JNK proteins,78 thereby activating these kinases in response to environmental stress (e.g., UV and γ-irradiation, heat shock, hyperosmolarity), peroxide exposure, pro-inflammatory cytokines, T-cell receptor stimulation, or developmental cues. In particular, it acts in dorsoventral patterning, convergent extension, and somitogenesis [588]. It targets P38MAPKs and JNKs. Phosphorylation sites of MAP2K4 are Ser257 and Thr261 [267].79 Enzymes MAP3K1 to MAP3K5, MAP3K7, MAP3K8, and MAP3K10 to MAP3K12 can activate MAP2K4 enzyme.
Enzyme MAP2K4 also interacts with MAP4K2, MAP2K7 (or JN2K2), MAPK8 (or JNK1), MAPK-interacting proteins MAPK8IP3 and MAPK8IP4,80 PKB, arrestin-β2, actin-binding filamins FlnA and FlnC,81 and nephrin [251]. Anti-apoptotic MAP2K4 protects from excessive TNFSF6-stimulated apoptosis, although this process eliminates autoreactive cells that can appear during early stage of T- and B-lymphocyte development [267].
6.6.5 MAP2K5
Enzyme MAP2K582 is a dual-specificity protein kinase. It is the single identified component of the MAPK set that targets MAPK6 (a.k.a. MAPK7, ERK4, and ERK5).
Some splice variants of MAP2K5 generated by reading frame shift can have different subcellular locations: the long isoform (MAP2K5L) localizes to the cytoskeleton, whereas the short isoform (MAP2K5S) remains in the cytosol [267]. A second splicing event (in- or exclusion of the 5′ alternative splice site) yields MAP2K5α (448 amino acids) , which is restricted mostly to the brain and liver, and ubiquitous MAP2K5β (359 amino acids) isoforms. Both MAP2K5α and MAP2K5β have 2 isoforms according to in- or exclusion the 3′ alternative splicing site, i.e., without or with a deletion of 10 amino acids in the catalytic domain. Full-length MAP2K5 contains the PB1 domain83 that enables interaction with its partners, such as MAP3K2, MAP3K3, and protein kinase-Cζ [589].
Phosphorylation sites of MAP2K5L are Ser311 and Thr315. Enzymes MAP3K2, MAP3K3, and MAP3K8 can activate MAP2K5 [251, 267]. Enzyme MAP2K5 also interacts with itself and protein kinase-Cζ [251]. Muscle-specific A-kinase anchoring protein AKAP6 connects to both MAP2K5 and ERK5 enzymes, thereby inhibiting PDE4d3 phosphodiesterase. In addition, RapGEF3 can be recruited by the AKAP6–PDE4d3 inhibits ERK5 activation.
6.6.6 MAP2K6
Enzyme MAP2K684 is a ubiquitous dual-specificity protein kinase85 highly selective for P38MAPK enzymes in a cell- and context-specific manner. It strongly activates all known P38MAPK isoforms. It is activated by all identified P38MAPK stimuli. In particular, upon DNA damage and cell stresses, MAP2K6 phosphorylates P38MAPKα and provokes its nuclear translocation. The ability to phosphorylate and activate P38MAPKs is shared with MAP2K3 and MAP2K4, but with a non-redundant and selective manner in response to specific stimuli [590].
The Map2k6 transcript generates alternatively spliced variants in humans.86 Its phosphorylation sites are Ser207 and Thr211 [267]. Enzymes MAP3K1, MAP3K3 to MAP3K5 (ASK1), MAP3K7 (TAK1), MAP3K8, and MAP3K17 (TAOK2) can activate MAP2K6 enzyme.87 Enzyme MAP2K6 abounds in the skeletal muscle, where it promotes myogenic differentiation using the P38MAPK pathway [590].
Protein MAP2K6 also interacts with MAP2K3, phospholipase-Cβ2, inhibitor SMAD7, and nephrin [251]. It also associates with microtubules and proteins from the transport machinery such as dynactin [590].
Protein Ser/Thr kinase C-related kinase PKN1 (PKNα, or PAK1) phosphorylates (activates) MAP3K15 that phosphorylates (activates) MAP2K6 enzyme. This fatty acid- and Rho-activated kinase may also act as a scaffold for MAP2K6–P38MAPKγ signaling [590].
6.6.7 MAP2K7
Enzyme MAP2K788 is a crucial transducer upstream from JNK signaling, in addition to MAP2K4 enzyme. It has a larger affinity for JNK enzymes.89 It phosphorylates specific Tyr and Thr residues in the activation loop of JNK proteins, thereby activating these kinases in response to environmental stress (e.g., UV and γ-irradiation, heat shock, hyperosmolarity), peroxide exposure, pro-inflammatory cytokines, T-cell receptor stimulation, or developmental cues. In particular, it acts in dorsoventral patterning, convergent extension, and somitogenesis [588].
Enzyme MAP2K7 is strongly activated by cytokines TNF and IL1.90 Enzyme MAP3K1 can activate MAP2K7 isoforms (MAP2K7α1–MAP2K7α2, MAP2K7β1 –MAP2K7β2, and MAP2K7γ1).91 Enzyme MAP3K3 can activate MAP2K7α1 and, to a much lesser extent, MAP2K7α2, MAP2K7β1, and MAP2K7β2. Enzymes MAP3K11 and MAP3K12 can stimulate MAP2K7 (strongly MAP2K7β1 and MAP2K7β2, moderately MAP2K7α isoforms). Enzyme MAP3K10 can activate MAP2K7α1.
Enzyme MAP2K7 also interacts with affectors MAP3K2, MAP3K5, MAP3K11, MAP3K12, MAP3K15, and MAP2K4 (or JN2K2); effectors MAPK8 (or JNK1) and MAPK14 (or P38MAPKα); MAPK-interacting proteins MAPK8IP1 to MAPK8IP3; dual-specificity phosphatase DuSP22; and growth arrest and DNA-damage-inducible GADD45β [251].
6.7 Mitogen-Activated Protein Kinases
The MAPK cascades include in their downstream tier several intracellular signaling networks. The 3 main MAPK cascades involved in signal transduction include (Table 6.17): (1) extracellular signal-regulated protein kinases, with its 2 isoforms ERK1 and ERK2; (2) Jun N-terminal kinases (JNK), with its 3 components JNK1 to JNK3; and (3) P38MAPK isozymes. The 2 other MAPK families are ERK3 and ERK4. The former is mainly regulated by autophosphorylation. The latter is activated by growth factors; it is required for cardiovascular development.
Table 6.17
Types of mitogen-activated protein kinases (ERK: extracellular-signal-regulated kinase; JNK: Jun N-terminal kinase; SAPK: stress-activated protein kinase).
MAPK type | Other aliases |
---|---|
MAPK1, MAPK2 | ERK2 |
MAPK3 | ERK1 |
MAPK4, MAPK5, MAPK12 | ERK3, ERK6, P38MAPKγ |
MAPK6, MAPK7 | ERK4, ERK5 |
MAPK8 | JNK1, SAPKγ |
MAPK9 | JNK2, SAPKα |
MAPK10 | JNK3, SAPKβ |
MAPK11 | P38MAPKβ |
MAPK13 | P38MAPKδ |
MAPK14 | P38MAPKα |
MAPK15 | ERK7, ERK8 |
Enzymes of the ERK, JNK, and P38MAPK subfamilies phosphorylate both transcription factors (Table 6.18) and other protein kinases. Whereas certain substrates, such as MAPK-activated protein kinases MAPKAPK2, MAPKAPK3, and MAPKAPK5 (or PRAK), are selectively recruited by stress-activated MAPKs, MAPK-interacting Ser/Thr kinases MNK1 and MNK2 as well as mitogen- and stress-activated protein kinases MSK1 and MSK2 are activated by both stress- and mitogen-regulated MAPKs and, like transcription factor AP1, integrate both stress and mitogenic signalings (Table 6.19) [267].
Table 6.18
Transcription factors targeted by MAPKs (Source: [267]; NFAT: nuclear factor of activated T cells). Phosphorylation of Jun is exclusively catalyzed by JNKs.
MAPK | Stimulus | Transcription factors |
---|---|---|
ERK1/2 | Mitogens | ELk1/4, Fos |
ERK4 | Mitogens | MEF2a/c, Jun |
(ERK5) | Stress, cytokines | |
JNK | Mitogens | Jun |
Stress, cytokines | ATF2 | |
ELk1/4, Fos | ||
NFAT2, NFAT4 (inhibition) | ||
P38MAPK | Stress, cytokines | ATF2 |
ELk1/4, Fos | ||
NFAT2, NFAT4 | ||
MEF2a/c, Jun | ||
GADD153 | ||
Max |
Table 6.19
MAPK targets of the RSK subfamily (RSKi: ribosomal protein S6 kinase, 90-kDa polypeptide-i, i = 1, …, 5).
Type | Gene | Alias in the MAPK superfamily |
---|---|---|
RSK1 | RPS6KA1 | MAPKAPK1a |
RSK2 | RPS6KA3 | MAPKAPK1b |
RSK3 | RPS6KA2 | MAPKAPK1c |
RSKb | RPS6KA4 | MSK2 |
RLPK | RPS6KA5 | MSK1 |
The MAPK enzymes target some transcription factors. All the MAPKs — ERKs (mainly in response to mitogens), ERK4 (primarily in response to stress), JNKs, and P38MAPKs — contribute to activity of AP1 activator protein by stimulating the transcription of genes that encode AP1 components.92 In resting cells, Jun is phosphorylated by glycogen synthase kinase GSK3 or CK2 casein kinase. Once cells are stimulated, GSK3 is inactivated via the PI3K axis. Conversely, phosphorylated Jun or ATF2 is active. Members of the JUN subfamily can be activated by JNKs (Jun: Ser63 and Ser73; JunD: Ser90 and Ser100). On the other hand, members of the ATF category are phosphorylated by both JNKs and P38MAPKs (ATF2: Thr69 and Ser71). Promoter of the FOS gene possesses a serum response element (SRE) that links to a heterodimer made of serum response factor (SRF) and a member of the ternary complex factor (TCF) family (e.g., ELk1 and ELk4). Enzymes of the JNK and ERK subfamilies, but not P38MAPKs, phosphorylate ELk1 (Ser383 and Ser389), whereas P38MAPKs phosphorylate nuclear transcriptional stimulator SAP1a (Ser381 and Ser387), or ELk4, to enhance the SRF–TCF connection, thereby initiating SRE activation. Enzymes ERK4 and P38MAPKs also phosphorylate some transcription factors of the myocyte enhancer factor MEF2 group (MEF2a–MEF2d) that target the JUN promoter.93
Transcriptional factor DNA-damage-inducible transcript DDIT394 represses certain cAMP-regulated genes and activates some stress-induced genes. Nuclear factor of activated T cells, such as NFAT2 (NFATc1) and NFAT4 (NFATc3), that are phosphorylated by JNKs are sequestered in the cytosol. Dephosphorylation (activation) of NFAT by PP3 allows nuclear translocation and DNA binding. Enzyme MAP3K1 can prevent NFAT4 dephosphorylation [267]. The class-D basic helix–loop–helix peptide MYC associated factor-X (MAX)95 that can complex with P38MAPKα interacts with Myc transcription factor that regulates cell proliferation, differentiation, and apoptosis.
The family of calcium–calmodulin-dependent protein kinases, which act downstream from mitogen-activated protein kinases, includes: (1) members of the ribosomal S6-kinase family with the mitogen- and stress-activated kinase infrafamily (MSK1 and MSK2; i.e., RLPK and RSKb) and MAPKAPK1 infrafamily (RSK1–RSK3); (2) MAPK-activated protein kinases (MAPKAPK or MK; i.e., MK2, MK3, and MK5); and (3) MAPK-interacting kinases (MNK1–MNK2) (Table 6.20). Enzymes of the RSK set are exclusively activated by extracellular signal-regulated kinases. Enzymes of the MSK and MNK categories are downstream effectors of both ERK and P38MAPK proteins. Enzymes of the RSK and MSK sets regulate gene expression, as they both phosphorylate Fos on the one hand, and cAMP-responsive element–binding proteins or histone-3, respectively, on the other. Stabilization of mRNA induced by P38MAPKs is mediated by MAPKAPK2 [267]. The JNK enzymes can also stabilize mRNA via MAP3K1 and MAP2K7 enzymes.
Type | Substrates |
---|---|
ERK1 | MAPKAPK1, MNKs, MSKs |
ERK2 | MAPKAPK1, MNKs, MSKs |
P38MAPKα | MAPKAPK2/3, MSKs |
P38MAPKβ | MAPKAPK2/3, MSKs |
Enzymes of the MNK set phosphorylate eukaryotic translation-initiation factor eIF4e and factors that bind to certain mRNAs, to regulate the expression of a specific category of proteins. These kinases are particularly involved in growth control and inflammation. Kinase MK2, activated by P38MAPK, is required for cytokine synthesis in inflammation and the cell cycle. Enzyme MK3, also activated by P38MAPK, regulates chromatin remodeling. Protein MK5, which particularly interacts with ERK3, is activated by ERK and P38MAPK, but not JNK kinases.
6.7.1 JNKs and P38MAPKs in Cardiovascular Diseases
Enzymes of the JNK and P38MAPK subfamilies, via MAP3K6 and MAP3K7, contribute to maladaptive cardiomyocyte hypertrophy in response to pressure overload. In addition, vascular wall hypertension elicits the release of the vasoactive peptides angiotensin-2 and endothelin-1 that also cause cardiomyocyte hypertrophy (Vol. 5 – Chap. 6. Heart Wall). Enzymes of the JNK and P38MAPK subfamilies participates in ischemia–reperfusion injury (Vol. 6 – Chap. 6. Heart Pathologies), in particular via MAP3K3 that preferentially recruits P38MAPKα and P38MAPKβ. Enzyme MAP3K3 also regulates the development of the cardiovascular apparatus via the P38MAPK pathway and MEF2c transcription factor.
6.7.2 Family of Extracellular Signal-Regulated Kinases
6.7.2.1 ERK Signaling Duration and Spatial Organization
ERK Signaling Duration and Cell Fate
Signaling duration contributes to the control of cell responses to stimulated signaling pathways that share signaling modules. Transient activation with negative feedback of extracellular signal-regulated kinases by epidermal (EGF), keratinocyte (KGF1, or FGF7, and KGF2, or FGF10), and insulin-like (IGF1) growth factors can cause cell proliferation [591]. On the other hand, sustained ERK activation with positive feedback by nerve growth factor and heregulin can provoke cell differentiation, whereas that by epidermal and hepatocyte growth factor can induce cell migration.
ERK Subcellular Localization and Scaffolding
A scaffold can stabilize preformed assemblies of signaling effectors as well as regulate the specificity and magnitude of signaling. Stimulation by epidermal- and nerve growth factor releases ERK from preformed complexes. In addition, nerve growth factor provokes ERK nuclear translocation. In the nucleus, ERK phosphorylates transcription factors and primes the export of transcriptional inhibitors. In the cytosol, ERK controls compartmentation of signaling mediators. Nerve growth factor induces the sustained dissociation of RasGAP neurofibromin NF1 from a Ras–ERK complex, thereby avoiding rapid Ras and subsequent ERK inactivation. Furthermore, NGF causes a sustained liberation of ERK from 15-kDa phosphoprotein enriched in astrocytes (PEA15) that sequesters ERK in the cytosol. Signaling based on ERK localizes to different subcellular compartments according to distribution of ERK partners that also serves as substrates.
Fully vs. Partially Scaffolded Signaling Complexes
Protein MAPK organizer MOrg196 enables the assembly of various signaling complexes with distinct outcomes. Scaffold MOrg1 can actually interact with Raf, MAP2K, and ERK (fully scaffolded complexes) as well as MAPK scaffold protein MAPKSP197 that recruits both MAP2K and ERK (partly scaffolded complexes) [591].
Scaffold Types and Subcellular Localization
Different scaffold proteins are used in different intracellular sites to target ERK substrates. Rapid and transient ERK activation and nuclear translocation is triggered from the plasma membrane by GPCRs, protein kinase-C, and Src kinase, as well as receptor Tyr kinases such as EGFR. On the other hand, sustained ERK signaling that is restricted to the cytosol is primed from internalized GPCRs in endosomes by β-arrestin and Raf kinase. β-Arrestin associates with entire MAPK modules, such as Raf–MAP2K–ERK and MAP3K5–MAP2K4–JNK3 modules. Discrimination among MAPK modules can be supported by cell locus (e.g., distinct Ras nanoclusters at the plasma membrane), context, and affector and partner types, such as kinase suppressor of Ras that serves as a scaffold for the Raf–MAP2K–ERK module.98 Therefore, ERK signaling triggered by GPCRs evolves into 2 phases: (1) an early signaling from the plasma membrane with ERK nuclear translocation followed by (2) a signal emission during endocytosis with ERK signaling confined to the cytosol. Signal integration from dually phosphorylated ERK results from combination of nuclear and cytosolic ERKPP concentrations.
The specificity of ERK substrate depends on the location of upstream mediators. Scaffolds participate in the determination of ERK substrates in the cytosol or nucleus according to Ras activation foci. At the plasma membrane, kinase suppressor of Ras facilitates the phosphorylation of cytoplasmic phospholipase-A2 by ERK [591]. On the cytoskeleton, the negative ERK feedback that is mediated by scaffold IQ motif-containing GTPase-activating protein IQGAP1 phosphorylates epidermal growth factor receptor at the plasma membrane. On the Golgi body membrane, interleukin-17 receptor-D also promotes phosphorylation by ERK of cytoplasmic PLA2 that generates arachidonic acid, a precursor to leukotrienes and prostaglandins.
Spatiotemporal Dynamics of ERK Signaling
Spatiotemporal network dynamics govern cell fate decisions. Computational models exhibit distinct modes of ERK spatiotemporal dynamics according to feedback type from active, dually phosphorylated ERK to Raf kinase or its regulators, such as Raf kinase inhibitor protein (RKIP) and Ras-activating guanine nucleotide-exchange factor Son of sevenless, and kinetic parameters [591]: (1) monotone, sustained response; (2) transient, adaptive output; (3) damped and (4) sustained oscillations; and (5) bistable outcome, in which 2 switch-like,stable steady states coexist. Different ERK temporal responses can also be observed experimentally.
Signaling Termination and Phosphatases
Signaling from ERK kinases causes negative feedback using Ser/Thr, Tyr, and dual-specificity phosphatases (Sect. 8.3.13.3). The latter dephosphorylate ERK1, ERK2, JNKs, and P38MAPKs. They are encoded by immediate-early genes, i.e., genes that are stimulated rapidly and do not require new protein synthesis for their transcription. Nevertheless, the time scale for DUSP-mediated feedback is longer than that of negative feedback caused by already synthesized inhibitors. Dual-specificity phosphatase isoforms localize to the cytoplasm and nucleus, hence regulating both cytoplasmic and nuclear ERK pools.
6.7.2.2 ERK1 and ERK2 Infrafamily
Extracellular signal-regulated kinases ERK1 and ERK2 are expressed in many tissues. They are activated by growth factors to regulate cell adhesion, growth, proliferation, differentiation, migration (during both chemotaxis and haptotaxis), and apoptosis. In particular, activated ERK1 and ERK2 regulates membrane protrusions and focal adhesion turnover by phosphorylating (activating) myosin light-chain kinase and calpain. They also regulate focal adhesion dynamics by influencing the interaction between paxillin and focal adhesion kinase. Unlike adhesion signaling, growth factors activate both MAP2K1 and -2.
The corresponding multipotent pathway achieves response specificity especially owing to interactions with scaffold proteins. Scaffolds include kinase repressor of Ras, β-arrestin, IL17Rd, and IQGAP1 proteins. Molecule KSR produces a docking platform at the plasma membrane. Agent IQGAP1 links the MAP2K–ERK pathway to the cytoskeleton.
In unstimulated cells, scaffold protein KSR1 prevents improper ERK cascade activation by sequestering MAP2K away from Raf kinase. In response to growth factors, KSR1 that localizes to the plasma membrane contributes to the spatiotemporal control of the Raf–MAP2K–ERK signaling. Both MAP2K1 and MAP2K2 kinases possess a KSR-binding motif that allows the formation of the bRaf–MAP2K–KSR ternary complex [592]. Docking of active ERK to KSR1 scaffold allows ERK to phosphorylate KSR1 and bRaf, hence ensuring a feedback control. Phosphorylation of KSR1 and bRaf actually promotes their dissociation and causes KSR1 release from the plasma membrane. Therefore, KSR1 first potentiates signal transmission, as it colocalizes cascade components to facilitate Raf–MAP2K interaction, thereby increasing efficiency and specificity of signaling, and then attenuates ERK activation, thereby controlling intensity and duration of ERK signaling.
The Ras–Raf–MAP2K–ERK signaling cascade triggered by growth factors and intercellular contacts controlled by IQGAP family proteins controls cell proliferation. Protein IQGAP3 is specifically expressed by proliferating cells to cooperate with Ras GTPase in the cell cycle [593].
Phosphoprotein-enriched in astrocytes PEA15 binds ERK and RSK2 for sequestration in the cytoplasm. Protein RSK2 is an ERK substrate kinase that can activate CREB-mediated transcription. Protein PEA15 independently binds ERK and RSK2 and enhances ERK binding to and phosphorylation (activation) of RSK2 in a concentration-dependent manner [594].
Endosome location of the MAP2K1–ERK pathway is required for full activation of ERK. Adaptor LAMTOR199 anchored to membrane rafts of late endosome connects late endosomes to the MAP2K1–ERK pathway via the LAMTOR2–MP1 complex that serves as a scaffold for MAP2K1 [595].100 LAMTOR1 is required for vesicle dynamics that comprise Rab11-mediated endosome recycling and lysosome processing, thus participating in the regulation of vesicular interactions and material transport along the cytoskeleton.
Enzymes of the MAP3K set in the ERK1 and ERK2 cascades101 are usually members of the RAF family (aRaf–cRaf) that bind to Ras GTPase to be activated. Upstream activators bind to receptor Tyr kinases or G-protein-coupled receptors. Activation of the ERK pathway can result from stimulation of plasmalemmal proteins Ras via receptor Tyr kinases in association with GRB2 adaptor and Son of sevenless, a guanine nucleotide-exchange factor. Activation of Ras triggers Raf recruitment to the plasma membrane and stimulation, phosphorylation (activation) of MAP2K1 and/or MAP2K2, and then activation of ERK1 and ERK2 kinases. Enzymes ERK1 and ERK2 have many targets, such as transcription factors (NFκB, Jun, and ELk1), kinases (MAPKAPK1 and MAPKAPK2), plasmalemmal receptors (EGFR), upstream MAPK tier activators (cRaf), SOS affector, and paxillin.
Recruitment of scaffolds and adaptors, phosphorylation, and dephosphorylation yields temporospatial regulation of the ERK pathway. Upon activation by growth factors and other stimulators such as urokinase plasminogen activator of the Ras–Raf–MAP2K1/2–ERK1/2 cascade, Enzymes MAP2K1 and MAP2K2 are phosphorylated by kinase Raf (Ser218 and Ser222 of MAP2K1 and Ser222 and Ser226 of MAP2K2) and phosphorylate substrates ERK1 and ERK2 (on specific tyrosine and threonine residues). The Ras–Raf–MAP2K1/2–ERK1/2 cascade initiates not only a rapid signal amplification, but also a negative feedback loop via MAP2K1/2 heterodimer formation and ERK-mediated phosphorylation of MAP2K1 [597]. Agent MAP2K1 restricts Raf-induced MAP2K2 phosphorylation. Enzyme ERK can also exert negative feedback on several upstream components of the pathway, such as SOS, PAK, cRaf, and bRaf.
Homo- and heterodimerization are common events in ERK signaling. In particular, bRaf and cRaf can form heterodimers with stronger kinase activity than that of monomers or homodimers [598]. Heterodimerization is enhanced by proteins 14-3-3 and by mitogens independently of ERK. Phosphorylation by ERK of bRaf promotes Raf heterodimer disassembly.
Nearly half of ERK1 and ERK2 are bound to microtubules with a role in polymerization dynamics. Kinases ERK1 and ERK2 also localize to adherens junctions (intercellular contact) and focal adhesions (cell–matrix contact), thereby operating in cell motility. Enzymes ERK1 and ERK2 also reside in the nucleus. In the cytoplasm, they can phosphorylate certain transcription factors prior to nuclear entry. Proteins that alter the subcellular localization of ERK1 and ERK2 can generate diseases [599].
Protein kinase-C can activate cRaf, and hence plays a role at the MAP4K stage. In endothelial cells, activation of ERK1 and ERK2 that depends on shear stress102 relies on PKCε [600].103 Affector cRaf, an ERK1/2 activator, is recruited to the plasma membrane, and activated by Ras, possibly phosphorylated by CSK on the one hand and by diacylglycerol-regulated PKCα and PKCε on the other hand [601].
Protein kinases contains a site aimed at selectively binding nucleotides and inhibitors. Inhibitors prevent autophosphorylation. A gatekeeper residue in ERK2 impedes auto-activation [603].104
Urocortin (Ucn), a member of the corticotropin-releasing factor family and its homologs urocortin-3, or stresscopin (Scp), and urocortin-2, or stresscopin-related peptide (SRP), are potent cardioprotectors in cells exposed to hypoxia–reoxygenation events. Whereas urocortin binds to both types of CRF G-protein-coupled receptors, CRFR1 and CRFR2, urocortin-2 and -3 tether exclusively and with high affinity to CRFR2. However, all 3 peptides activate the ERK1/2 and PKB pathways [604].
The signaling adaptor sequestosome-1 regulates osteoclastogenesis via ubiquitin ligase TRAF6 and activation of nuclear factor-κB. It also promotes Ras-induced cell transformation. Moreover, sequestosome-1 impedes adipogenesis and insulin resistance, without alterations in feeding or locomotion, but favors energy combustion [605]. Sequestosome-1 sequesters extracellular signal-regulated kinase ERK1 into cytoplasm in adipose tissue. Its synthesis is induced during adipocyte differentiation (negative feedback) to prevent excessive adipogenesis. Ribosomal S6 kinase is an ERK substrate in adipogenesis.
6.7.2.3 ERK3 Subfamily: ERK3 (or ERK6) and ERK4 (or ERK5)
Extracellular signal-regulated kinase-3 (ERK3)105 and -4 (ERK4)106 define a distinct subfamily of MAPKs, the ERK3 subfamily, that exists exclusively in vertebrates [606].
ERK3(6)
ERK4(5)
Extracellular signal-regulated kinase ERK4, or ERK5, like ERK1 and ERK2, is activated by growth factors. Hence, it participates in the regulation of cell proliferation, differentiation, and migration. especially during the development of the cardiovascular system and neural differentiation (Table 6.21). It is also stimulated by environmental stresses, such as oxidative stress (peroxide) and hyperosmolarity (sorbitol). In adults, ERK4 is mostly expressed in heart, brain, and lung [606].
Table 6.21
Affectors and effectors of ERK5 compared to those of ERK1 and ERK2 (Source: [607]; AKAP6: mAKAP [muscle-selective A-kinase anchoring protein]; PEA15: 15-kDa phosphoprotein enriched in astrocytes; SGK: serum- and glucocorticoid-regulated kinase). The MAP2K5–ERK5 axis regulates cell proliferation, apoptosis, and inflammation.
ERK5 (ERK4) | ERK1 and ERK2 |
---|---|
Stimuli | |
Nerve growth factor | Nerve growth factor |
Oxidative stress | Platelet-derived growth factor |
Hyperosmolarity | |
Transcription factors | |
ELk1 | Myocyte enhancer factor MEF2 |
MyC | MyC |
Fos, Fra1, Jun | Fos, Jun |
PPARγ1 | |
Kinases | |
Ribosomal S6 kinase | Ribosomal S6 kinase |
SGK | |
Miscellaneous | |
SAP1a | SAP1a |
Cyclin-D1 | Cyclin-D1 |
AKAP6 | PEA15 |
Sef | |
Effects | |
Cardiovascular development | Embryogenesis (MAP2K1–ERK2) |
Neural differentiation | Mesoderm formation |
Nucleocytoplasmic transport | |
Nuclear localization signal | Passive diffusion (monomer) |
Nuclear export signal | Active transport (dimer) |
Interaction with nuclear-pore complex |
Enzyme ERK4 is required in endothelial cells, as its absence in endothelial cells, but not cardiomyocytes, causes cardiovascular defects. The N-terminus of ERK4 contains the kinase domain that is similar to that of ERK1 and ERK2 kinases.
The C-terminus of ERK4 enables its coactivator-like action aimed at increasing the transcriptional activity of some of its targets. Protein ERK4 actually is a kinase that has transcriptional activity due to its long C-terminus, independently of its kinase activity. Therefore, ERK4 can transmit signals from MAP2K5 to its effectors by phosphorylation or transactivating specific transcription factors, such as peroxisome proliferator-activated receptors PPARγ and PPARδ and myocyte enhancer factor MEF2 (MEF2a, MEF2c, and MEF2d, but not MEF2b) [589]. The latter activity is precluded by reactive oxygen species and advanced glycation end product (AGE)-dependent sumoylation.108
Enzymes MAP3K2 and MAP3K3 activate MAP2K5, a specific kinase for ERK4 kinase. The ERK4 signaling leads to ERK4 translocation into the cell nucleus. Activated ERK4, like ERK1 and ERK2, stimulates Fos and Jun factors. It promotes the expression of cyclin-D1, which regulates the G1–S transition of the cell cycle, implicating a cAMP response element [607].
Kinase ERK4 phosphorylates (activates) serum response element via ETS domain-containing transcriptional factor ELk4,109 but not via the related ELk1 transcription factor [589]. It phosphorylates Fos at its nuclear export signal (Thr232), hence preventing Fos egress, and binding motif (Ser32), thereby precluding the interaction with ubiquitin ligase component N-recognin-1 (UbR1).110 Kinase ERK4 can support transactivation of Fra1, a member of the FOS subfamily (with Fos, FosB, and Fra2). In endothelial cells, ERK5 operates via Krüppel-like factor KLF2 to counteract endothelial cell activation that occurs in response to pro-inflammatory stimuli [589].
6.7.3 Family of Jun N-Terminal Kinases
The family of Jun N-terminal kinase (JNK), or stress-activated protein kinase (SAPK) regulates stress responses, neural development, inflammation, and apoptosis. In mammals, the JNK family comprises 3 proteins encoded by 3 distinct genes (Jnk1–Jnk3), transcripts of which generate 10 splicing variants. Two isoforms JNK1 and JNK2 are ubiquitous, whereas tissue-specific isoform JNK3 lodges in the brain, heart, and testis.
The MAP3K enzymes that target the JNK pathway include mixed lineage kinases (MLK1–MLK3 and DLK, i.e., MAP3K9–MAP3K12), MAP3K1, and MAP3K5. Many involved MAP3Ks are activated by Rho GTPases. These MAP3Ks activate MAP2K4 and MAP2K7 enzymes.
The specificity of JNK signaling results, partly, by the formation of distinct JNK signaling complexes with JNK and JNK interactors, such as MAP2K4 and MAP2K7 enzymes and various scaffold proteins. The latter can assemble functional multienzyme modules that encompass a MAP3K, a MAP2K, and a MAPK. These scaffold proteins bind specifically to different JNK isoforms and different MAPKs and MAP2Ks for signaling insulation (Vol. 3 – Chap. 1. Signal Transduction) and proper spatiotemporal sites.
The JNK enzymes are regulated by multiple scaffolds, JNK-interacting proteins (JIP1–JIP4; Table 6.22), or MAPK8-interacting proteins (MAPK8IP1–MAPK8IP4), as well as β-arrestin-2, filamin,112 and CRK2, for kinase activation and/or substrate selection. Other scaffold proteins involved in JNK signaling modules comprise SH3 domain-containing ring finger protein SH3RF1.113
Table 6.22
Scaffold proteins, especially JNK-interacting proteins (JIP), or MAPK8-interacting proteins (MAPK8IP), confer specificity to MAP2K activity (Source: [588]; MAX: MyC-associated factor-X). Scaffold proteins JIP1, JIP2, and JIP3 can form homo- and hetero-oligomers. They can thus connect 2 distinct sets of signaling modules, one containing MAP2K4, and the other incorporating MAP2K7 protein. In addition, MAP3K enzymes can act as scaffolds in addition to their intrinsic kinase activities.
Type | Other alias | Interactors |
---|---|---|
JIP1 | MAPK8IP1 | Various MLKs (e.g., MAP3K9–MAP3K11), MAP2K7, JNK |
JIP2 | MAPK8IP2 | Various MLKs (e.g., MAP3K9–MAP3K11), MAP2K7, JNK |
JIP3 | MAPK8IP3 | MAP3K1, various MLKs, MAP2K4/7, JNK |
JIP4 | MAPK8IP4 | MyC–JIP4–MAX, JNK–JIP4–MAP3K3, |
JNK–JIP4–MAP2K4, JNK–JIP4–P38MAPK complex |
The JNK enzymes phosphorylate numerous transcription factors, such as Jun, ATF2, ELk1, P53, and Myc, as well as other proteins, such as BCL2, BCLxL, paxillin and microtubule-associated proteins (e.g., MAP1b and MAP2). Diverse splice variants of the 2 ubiquitous JNK1 and JNK2 isoforms and tissue-specific JNK3 isoform display different selectivity and affinity for target transcription factors. Subtypes of JNK2 have higher affinity for Jun than JNK1 and JNK3 isozymes. Subtype JNK2α1 binds more strongly to Jun than JNK2β2 kinase. The JNK2α isoforms bind more tightly to Jun than ATF2, whereas JNK2βs bind more strongly to ATF2 than Jun [267].
Genotoxic stresses promote apoptosis, as they recruit JNKs and activate NFκB that, in turn, stimulates AP1 transcription factors. The JNK enzymes are activated in response to extracellular stimuli, such as pro-inflammatory cytokines TNF-α and IL1β.
6.7.3.1 JNK1 or MAPK8
Jun N-terminal kinase JNK1114 has various splice variants [267]: JNK1α1,115 JNK1α2,116 JNK1β1117 and JNK1β2.118
This major Jun N-terminal kinase is activated by most known JNK inducers and is responsible for most known JNK effects. It contributes to cell differentiation and proliferation. It also intervenes in TNFα-regulated activities, such as cell death and inflammation.119
Enzyme JNK1 activates Ub-ligase Itch of the HECT (NEDD4) family120 that specifically ubiquitinates the caspase-8 inhibitor Casp8 and FADD-like apoptosis regulator (CFLAR)121 to trigger its proteasomal degradation [608].
The pro-inflammatory cytokine tumor-necrosis factor-α exerts its pleiotropic activities via multiple effectors such as JNK1 kinase. Transcription factor Myc-interacting zinc finger protein-1 (MIZ1)122 that operates in transcriptional activation in the nucleus in response to cytoskeletal changes and impedes cell proliferation (transcriptional activator and repressor) also acts in the cytoplasm as a pathway-specific regulator, as it selectively suppresses TNFα-induced JNK1 activation and cell death [609]. Protein MIZ1 specifically regulates TNFα-induced TRAF2 Lys63-linked polyubiquitination. It, indeed, affects neither JNK1 activation by IL1β, nor TNFα-mediated activation of P38MAPK, ERK, and IκB kinase. Upon TNFα stimulation, MIZ1 is degraded rapidly by the proteasome to relieve its inhibition on JNK1 kinase.
Antigen-presenting cells, such as macrophages and dendritic cells, present antigen to naive T lymphocytes via T-cell receptors, CD3 subunit of which, in conjunction with CD28, initiates the TCR–CD28 costimulatory pathway. The latter promotes: (1) proliferation and maturation of CD4 + CD8 + cells to CD4 + TH lymphocytes that then become interferon-synthesizing TH1 and cytokine-producing TH2 effectors due to IL12 and IL4 as well as (2) production of some cytokines such as IL2. Lymphocytes TH1 produce interferon-γ and promote Ig switching in B lymphocytes, hence stimulating phagocytosis. On the other hand, TH2 cells produce IL4 and IL5 that induce switching to IgE and IgG4 in B lymphocytes that both bind to Fc receptor, either on mastocytes and basophils (IgE) or phagocytic cells (IgG4), and activate eosinophils, respectively. The TCR–CD28 costimulation generates a strong JNK1–AP1 activation [267]. Transcription factor AP1 is required for T-cell maturation and development of acquired immunity. However, JNK1 suppresses the differentiation of TH lymphocytes into TH2 cells, as it impedes NFAT1 activation and TCR-stimulated IL4 production, hence favoring TH1 cell differentiation.
6.7.3.2 JNK2 or MAPK9
The Jun N-terminal kinase JNK2123 has diverse splice variants [267]: JNK2α1,124 JNK2α2,125 JNK2β1,126 and JNK2β2.127 An additional isoform JNK2γ has been detected in human B lymphocytes.
Dimerization of JNK2 can support JNK2 activation. Nitrosylation and ubiquitination modify JNK2 activity. Extensive S-nitrosylation inhibits JNK2 phosphorylation [610].
Like all JNKs, JNK2 promotes cell death in various cell types. However, under some circumstances, JNK2 acts as an anti-apoptotic agent. Unlike ERK1 and ERK2, JNK2 is not strongly activated by mitogens, but vigorously by: (1) environmental stresses (heat shock, ionizing radiation, oxidative stress, DNA-damaging chemicals, reperfusion injury, shear stress, and protein synthesis inhibitors); (2) inflammatory cytokines of the TNF superfamily, such as TNF, interleukin-1, TNFSF5, TNFSF6, TNFSF7, TNFSF11a, etc.; and (3) vasoactive peptides, such as endothelin and angiotensin-2 [267]. According to the cell type and context, JNK2 is either preferentially activated by MAP2K4 or MAP2K7 [610].
Among JNK2 kinases, JNK2α1 and JNK2α2 have a very high affinity for Jun, whereas JNK2β1 and JNK2β2 link preferentially to Activating transcription factor ATF2. In addition, JNK2 phosphorylates P53 factor. It also activates nuclear factor of activated T-cells NFAT3 [610].
Enzyme JNK2 is involved in T-cell activation, as it enhances TCR–CD28 costimulation of T-cell proliferation and TCR-stimulated production of IL2, IL4, and Ifnγ by activated T lymphocytes [267]. Enzyme JNK2 also contributes to B-cell activation. It is also involved in the control of the helper TH1 and TH2 cells [610].
6.7.3.3 JNK3 or MAPK10
The Jun N-terminal kinase JNK3128 has several splice variants [267]: JNK3α1,129 JNK3α2,130 JNK3β1,131 and JNK3β2.132 It is predominantly expressed in the central nervous system. It is found, to a lesser extent, in the heart and testis. According to the context, JNK3 acts as a pro- or anti-apoptotic regulator; in particular, it preserves the pancreatic β-cell pool [611]. Unphosphorylated (inactive) JNK3 lodges mostly in the cytoplasm.
Protein JNK3 is phosphorylated (activated) by 2 dual-specificity kinases — MAP2K4 and MAP2K7 — (Thr221 and Tyr223). These activating kinases are themselves activated by MAP3K1 to MAP3K4 (MEKK family), MAP3K5 and MAP3K6 (ASK group), MAP3K7, MAP3K8, and MAP3K9 to MAP3K13 (MLK family). It is dephosphorylated (inactivated) by dual-specificity phosphatase MKP7 (or DuSP16) [611].
Scaffold proteins can facilitate the phosphorylation and dephosphorylation of JNK3 by linking to JNK3 and appropriate kinase(s) or phosphatase(s). These scaffolds include MAPK8-interacting proteins (MAPK8IP1–MAPK8IP3) [611]. Protein MAPK8IP1 aggregates MAP3K11, MAP2K7, and JNK3 enzymes to form a complete MAPK module. Scaffold β-arrestin-2 can selectively bind to JNK3 and assemble MAP3K5, MAP2K4, and JNK3 proteins.133 Both MAPK8IP1 and β-arrestin-2 can bind MKP7 (DuSP16) phosphatase. Phosphorylated MAPK8IP3 may interact with MAP2K4, MAP2K7, and JNK3 enzymes.134 Moreover, MAPK-binding protein MAPKBP1 (or JNKBP1) bind to JNK3, in addition to JNK1 and JNK2.
In addition, cyclin-dependent kinase CDK5 may phosphorylate (inactivate) JNK3 protein [611]. Cyclin-dependent kinase inhibitor CKI2a can also bind to JNK3, thereby suppressing its activity without affecting JNK3 phosphorylation. On the other hand, S-nitrosylation of JNK3 by NO can improve the phosphorylation level and activity of JNK3 kinase.
Activated JNK3 can phosphorylate transcriptional factors, such as Jun, ELk1, and ATF2 to increase the transcriptional activity [611]. Other substrates of JNK3 include P53, kinesin-1,135 microtubule-associated protein Tau (MAPT), stathmin-like 2,136 mitochondrial protein Second mitochondria-derived activator of caspases (SMAC),137 and Rab3 GDP–GTP-exchange factor (Rab3GEP).138
6.7.4 Family of P38 Mitogen-Activated Protein Kinases
The 38-kDa polypeptides — P38MAPKs — constitute a second group of stress-activated MAPK enzymes. Kinases of the P38MAPK family are activated by numerous factors: hormones, UV beams, ischemia, cytokines (e.g., interleukin-1 and tumor-necrosis factor), osmotic pressure changes, and heat stress.139 Almost all of these stimuli are able to recruit both JNK and P38MAPK kinases. During ischemia–reperfusion events, JNKs are activated during reperfusion, but not ischemia, whereas P38MAPKs are activated during ischemia, but not reperfusion. In addition, MAP2K3 and P38MAPK also contribute to TH1-cell development, as they provoke IL12 production by macrophages and dendritic cells.
Monomeric CDC42, Rac1, and RhoA GTPases are required for the re-entry of quiescent cells into the cell cycle G1 phase. On the other hand, in cells already committed to the cell cycle, CDC42, but not Rac1 or RhoA, targets P38MAPKs that can trigger cell cycle arrest at G1–S transition via MAP3K3 and MAP3K6 [267].
Four P38MAPK isoforms (P38MAPKα–P38MAPKδ) that are encoded by 4 distinct genes act in a cascade that involves MAP3Ks (MAP3K1–MAP3K4, ASKs, MLKs, DLK, TAK1, TAOKs, and TPL2, i.e., MAP3K5–MAP3K12 and MAP3K16–MAP3K18). These MAP3Ks activate MAP2K3, MAP2K4, and MAP2K6, which phosphorylate P38MAPK [612]. In the classic pathway (MAP3K–MAP2K cascade), P38MAPK is phosphorylated (activated) downstream from MAP2K3 and MAP2K6 enzymes. However, in some cells such as activated T cells, an alternative pathway exists for P38MAPK activation. Enzyme P38MAPK is phosphorylated by ZAP70, itself activated by SRC family kinase LCK (MAPK-independent P38MAPK activation) [613]. The P38MAPK enzymes are linked to scaffold proteins (JIP2, JIP4, and Osm).140
6.7.4.1 P38MAPKα or MAPK14
Enzyme P38MAPKα141 resides in both the cytoplasm and nucleus. In humans, 3 splice variants have been detected in addition to full-length P38MAPKα [614]: (1) cytokine suppressive anti-inflammatory drug-binding protein CSBP1; (2) exon skip Exip (as it skips exon 10); and (3) MAX-interacting protein MxI2, a shorter splice variant and a kinase that may interact with heterodimeric partner MyC-associated factor-X (MAX) of MyC transcription factor.
Enzyme P38MAPKα is required in erythropoietin synthesis and angiogenesis. It transmits chemotactic signals in neurons, endothelial and epithelial cells, neutrophils, mastocytes, monocytes, and vascular smooth muscle cells [614].
Its activation results from dual phosphorylation of the Thr180–Gly181–Tyr182 motif on the activation loop by MAP2K3, MAP2K4, and MAP2K6 enzymes (in vivo, mainly by MAP2K3 and MAP2K6, and under certain circumstances, by MAP2K4, an activator of JNKs). In addition to the these phosphorylation sites (Thr180 and Tyr182), P38MAPKα can be phosphorylated on Ser2, Thr16, and Thr241 [614].
Enzyme P38MAPKα is dephosphorylated (Thr180) by magnesium-depen-dent PPM1d protein phosphatase. Phosphatase PPM1a dephosphorylates MAP2K6 activator and P38MAPKα [614]. MAPK phosphatases, such as MKP1 (or DuSP1), MKP2 (or DuSP4), MKP5 (or DuSP10), and MKP7 (or DuSP16), and other dual-specificity phosphatases, such as DuSP2 and DuSP8, also dephosphorylate (inactivate) P38MAPKα.
Scaffold MAPK8IP2 binds to MAP3K11, MAP2K3, P38MAPKα, and TIAM1 (RacGEF) and RasGRF1 [614]. * In brown adipocytes, β-adrenergic receptor and protein kinase-A stimulate P38MAPKα via JIP2 that links to MAP2K3 activator. In addition, JIP4 (MAPK8IP4), which binds to, but does not activate JNK, supports MAP2K3- and MAP2K6-dependent activation of P38MAPKα, but does not bind MAP2K3 and MAP2K6, whereas it connects to MAP2K4 activator [614].
Cerebral cavernous malformation CCM2 homolog, or osmosensing scaffold for MAP3K3 (Osm), tethers to Rac, MAP3K3, and MAP2K3, and indirectly enables P38MAPKα activation in response to osmotic stress. In addition, MAP3K7IP1 (or TAB1) serves as a P38MAPKα binding partner. Last, but not least, P38MAPKα interacts with P38MAPK-interacting protein (P38IP) that regulates its activity.
Once it is activated by stress, it re-activates MAPKAPK2 kinase that phosphorylates heat shock protein HSP27 in response to interleukin-1β and LIMK1 kinase in response to VEGF in endothelial cells [614]. In addition, P38MAPKα targets caldesmon, an actin- and myosin-binding protein involved in the assembly of actin filaments, in smooth muscle cells, as well as paxillin, a phosphoprotein of focal adhesions [614].
Enzyme P38MAPKα participates in cell adaptation to stress, cell cycle regulation, inducing cell division arrest at various checkpoints. and control of cellular death (promotion or protection against cell death according to circumstances) [614].
Enzyme P38MAPKα is implicated in inflammation and its associated increase in vasculature permeability to enable influx of molecular and cellular mediators. It controls the production of COx2 cyclooxygenase and promotes prostaglandin synthesis, in particular in fibroblasts and endothelial cells [614]. It also regulates the synthesis of pro-inflammatory cytokines IL1 and TNFα in monocytes and neutrophils. It mediates the activation by distinct pattern-recognition receptors on airway epithelial cells.
Enzyme P38MAPKα is involved in viral and bacterial infections [614]. It provokes degranulation of neutrophils; in these cells, it also phosphorylates NADPH oxidase, thereby priming oxidative burst. In macrophages, P38MAPKα upregulates scavenger receptors for phagocytosis of bacteria in response to Toll-like receptor activation.
Enzyme P38MAPKα is involved in the activation of the transcription regulators of the superfamily-2B3A of nucleus-resident nuclear factors Activator protein AP1 and transcription factors of the ATF–CREB family,142 in conjunction with other MAPKs [614].143 In particular, G-protein-coupled receptor activates the Jun promoter via P38MAPKα and AP1 activation in monocytes depends on JNK and P38MAPKα. Moreover, in some circumstances, P38MAPKα enables activation of nuclear factor-κB.
6.7.4.2 P38MAPKβ or MAPK11
Signaling by P38MAPKβ144 depends on HDAC3, a class-1 histone deacetylase.145 During inflammation, the induction of immediate-early genes such as that of TNFα is regulated by signaling cascades, such as the JaK–STAT, NFκB, and P38MAPK pathways. Enzyme P38MAPKβ interacts directly and selectively with HDAC3 enzyme. This deacetylase decreases the P38MAPKβ phosphorylation state and inhibits the activity of the P38MAPKβ-dependent transcription factor Activating transcription factor ATF2 [615].146 Consequently, HDAC3 represses TNF gene expression, especially in monocytes and macrophages.
6.7.4.3 P38MAPKγ or MAPK12
Enzyme P38MAPKγ147 behaves as a stress kinase that can function independently of phosphorylation [616]. It intervenes in cell differentiation. In skeletal muscles, where it is highly expressed, it supports fusion capacity of myoblasts, thereby promoting formation from myoblasts of myotubes. Alone or together with P38MAPKα, it also acts in erythroid differentiation.
In addition, P38MAPKγ contributes to the regulation of the stress response and cell cycle. In response to hypoxia, P38MAPKγ is phosphorylated (Thr183 and Tyr185) [616]. It is activated by dual-specificity kinases MAP2K3 and MAP2K6 enzymes, as well as MAP3K8 and RhoA and Rit GTPases. Moreover, MAP3K15 (or MLK7) stimulates the MAP2K6– P38MAPKγ axis. On the other hand, it is dephosphorylated by MAPK phosphatase MKP1 (DuSP1) and cytoplasmic PTPn3 phosphatase [616].
Enzyme P38MAPKγ is activated by various cytokines, such as IL1 and TNFα, and stress types (e.g., hypoxia and osmotic stress) [616].
Enzyme P38MAPKγ efficiently phosphorylates ATF2, ELk1, ELk4, Fos, and P53 (Ser33), but weakly MEF2a, MEF2c, and MEF2d transcription factors [616].
It also phosphorylates microtubule-associated protein Tau and BTK-associated SH3 domain-binding protein SH3BP5, a mitochondrial JNK-interacting protein. Protein P38MAPKγ can also interact with estrogen receptor ERα (or nuclear receptor NR3a1) [616].
6.7.4.4 P38MAPKδ or MAPK13
Enzyme P38MAPKδ148 is involved in cell migration, in particular in invasion of carcinoma cells. In addition, P38MAPKδ pertains to the set of 13 lung-prominent gene products with vitamin-D-dependent calcium-binding protein S100g, solute carrier family transporter SLC29a1, corticotropin-releasing hormone receptor CRHR1, and lipocalin-2, a growth factor and component of the innate immune response to bacterial infection [617].
6.7.5 Stress-Activated Protein Kinases
Stress-activated149 protein kinases (SAPK) include JNKs and P38MAPKs activated by MAP3K1–MAP2K4/7 and MAP3K7–MAP2K3/6 cascades, respectively (Table 6.23). The MAP3K5 module stimulates both JNKs and P38MAPKs. Activated JNKs and P38MAPKs induce gene expression via different transcription factors, such as ATF2, ELk1, Jun, and MEF2.
Table 6.23
Stress-activated protein kinases.
Type | Other alias |
---|---|
SAPK1a, SAPKα | JNK2 |
SAPK-P46α1 | JNK2β1 |
SAPK-P46α2 | JNK2α1 |
SAPK-P54α1 | JNK2β2 |
SAPK-P54α2 | JNK2α2 |
SAPK1b, SAPKβ | JNK3 |
SAPK-P46β1 | JNK3β1 |
SAPK-P46β2 | JNK3α1 |
SAPK-P54β1 | JNK3β2 |
SAPK-P54β2 | JNK3α2 |
SAPK1c, SAPKγ | JNK1 |
SAPK-P46γ1 | JNK1β1 |
SAPK-P46γ2 | JNK1α1 |
SAPK-P54γ1 | JNK1β2 |
SAPK-P54γ2 | JNK1α2 |
SAPK2a | P38MAPKα |
SAPK2b | P38MAPKβ |
SAPK3 | P38MAPKγ |
SAPK4 | P38MAPKδ |
The SAPK molecules can be more or less implicated in cardiac remodeling associated with overload or ischemia–reperfusion injury, modulating signaling pathways associated with cardiac hypertrophy and cell apoptosis [618]. Enzyme P38MAPK can promote remodeling with interstitial fibrosis , leading to a loss in contractility, but without significant hypertrophy [619]. Cells can withstand relatively high mechanical stresses and react quickly and adapt using, at least partly, their dynamical cytoskeleton. Enzymes P38MAPK (but not ERK or JNK) are responsible for stretch-induced activation of an eicosanoid pathway that involves E-prostanoid receptor EP4 and cyclooxygenase COx2 (but not EP1 and COx1) via MAPKAPK2 [620]. However, prostaglandin-E2 induces actin filament depolymerization in cells stretched during a long time (6 h, 5% elongation, 0.5 Hz).
Downstream of mitogen-activated protein kinase pathways, MAPK-activated protein kinases regulate gene expression at the transcriptional and post- transcriptional levels and control the cell cycle. Moreover, MAPKAPKs are involved in actin remodeling, and consequently, in cell shape and cell motility [621]. Hsp27, an inhibitor of Rho-dependent actin stress fiber formation, competes with cofilin for binding to 14-3-3 protein. Increased release of cofilin from the 14-3-3 protein is followed by cofilin dephosphorylation (inactivation) and actin binding. Rho activation causes phosphorylation of cofilin by LIMK kinase and its release from the barbed ends of the actin filaments.
6.8 Kinase Substrates of MAPKs
All types of MAPKs, i.e., ERKs, JNKs, and P38MAPKs, phosphorylate various types of protein kinases: (1) MAPK-activated protein kinases (MAPKAPK); (2) MAPK-interacting Ser/Thr kinases (MNK); and (3) mitogen- and stress-activated protein kinases (MSK). Their stimulators, affectors, and effectors are exhibited in Table 6.24.
Table 6.24
Kinases as substrates of MAPKs (Source: [267]; MAPKAPK: MAPK-activated protein kinase; MNK: MAPK-interacting kinase; MSK: mitogen- and stress-activated protein kinase; CREB: cAMP-responsive element-binding protein; eIF: eukaryotic translation initiation factor; HSP: heat shock protein). Enzyme MSK1 can phosphorylate histone 3 and high-mobility group nucleosome-binding domain protein HMGN (or HMG14).
Kinase | Stimuli | Affector | Effector | Effect |
---|---|---|---|---|
MAPKAPK | Stresses | P38MAPK | HSP25/27 | Cytoskeleton organization |
Cytokines | P38MAPK | |||
MNK | Stresses | P38MAPK | eIF4e | Protein synthesis |
Cytokines | P38MAPK | |||
Mitogens | ERK | |||
MSK | Stresses | P38MAPK | CREB, H3, | Protein synthesis, |
Cytokines | P38MAPK | HMGN1 | chromatin remodeling | |
Mitogens | ERK | |||
RSK | Mitogens | ERK | H3 | Chromatin remodeling |
6.8.1 MAPK-Activated Protein Kinases
MAPK-Activated protein kinases correspond to members of the subfamily of P90 ribosomal S6 kinases (RSK1–RSK3; Table 6.25) that are Ser/Thr kinases activated by the ERK pathway. Other MAPKAPKs that are unrelated to the MAPKAPK1 infrafamily members form a small group of Ser/Thr kinases that include MAPKAPK2, MAPKAPK3 (also called three pathway-regulated kinase [3PK]), and MAPKAPK5 (or P38MAPK-regulated and activated protein kinase PRAK). In response to cellular stress and inflammatory cytokines, MAPKAPKs are phosphorylated (activated) by MAPKs.
Table 6.25
Group of type-1 MAPK-activated protein kinases that correspond to members of the P90RSK subfamily of ribosomal S6 kinases (RSK1–RSK3), in opposition to S6K enzymes of the P70RSK subfamily.
Type | Gene | Aliases |
---|---|---|
MAPKAPK1a | RPS6KA1 | RSK1, P90RSK1 |
MAPKAPK1b | RPS6KA3 | RSK2, P90RSK2, S6Kα3, MRX19, CLS, ISPK1, HU3 |
MAPKAPK1c | RPS6KA2 | RSK3, P90RSK3, S6Kα2, S6Kα, HU2 |
Enzyme MAPKAPK2 is phosphorylated (activated; Thr25, Thr222, and Ser272) by P38MAPKα and P38MAPKβ, but not P38MAPKγ or P38MAPKδ. Additional autophosphorylation (Thr334) follows. Enzymes MAPKAPK3 and MAPKAPK5 are also mainly phosphorylated by P38MAPKα and P38MAPKβ. Once they are activated, MAPKAPK2, MAPKAPK3, and MAPKAPK5 phosphorylate heat shock protein HSP27 (Ser15, Ser78, Ser82, and Ser90) to dissociate molecular chaperones HSP27 multimers into monomers and dimers and redistribute HSP27 to the actin cytoskeleton for its reorganization into stress fibers [267].
Like protein kinase-A, MAPKAPK1s and MAPKAPK2 target transcription factor cAMP-response element-binding protein (CREB). Yet, nuclear MSK1, but neither MAPKAPK1s nor MAPKAPK2, is the relevant stress- and mitogen-activated kinase for CREB factor.
6.8.2 MAPK-Interacting Ser/Thr Kinases
The MAPK-interacting Ser/Thr kinases MNK1 and MNK2 are phosphorylated (activated) by ERK1 and ERK2 in response to insulin and mitogens and P38MAPKs in response to stress. Kinases MNK1 and MNK2 phosphorylate eIF4e (Ser209). The eIF4f complex, i.e., the eIF4a–eIF4b–eIF4g–eIF4e complex, is involved in the recognition of the mRNA 5′-cap structure and recruitment of mRNA to ribosome. N7-methylguanosine-binding protein eIF4e recruits mRNAs onto scaffold eIF4g that also binds RNA helicase eIF4a. The latter, together with RNA-binding protein eIF4b, unwinds secondary structure in the mRNA 5′-untranslated segment to facilitate scanning of the mRNA by the 40S ribosomal complex to the ATG translational initiation site. Translational repressor 4E-binding protein 4eBP1150 is phosphorylated in response to insulin and mitogens to dissociate it from eIF4e that can then be incorporated into the eIF4f complex. Protein eIF4e is phosphorylated by target of rapamycin and other effector kinases of PI3K to raise its affinity for mRNA 5′-cap.
6.8.3 Mitogen- and Stress-Activated Protein Kinases
Mitogen- and stress-activated protein kinases MSK1,151 and MSK2152 constitute an infrafamily of Ser/Thr protein kinases. Kinase MSK1, a substrate of P38MAPKs, localizes to the cell nucleus. It is activated by mitogens and environmental stresses.
Enzyme MSK1 interacts with kinases MAPK1, MAPK11, and MAPK14, epidermal growth factor, transcription factor CREB1, and BCL2 antagonist of cell death (BAD) [251]. Enzyme MSK2 interacts with kinases MAPK3 and MAPK14 and transcription factors CREB1 and Fos [251].
Kinases MSK1 and MSK2 phosphorylate transcription factors cAMP response element (CRE)-binding protein CREB and cAMP-dependent ATF1 in fibroblasts in response to mitogens and cellular stress [622].153
Phosphorylation of CREB (Ser133) by MSK1 (with a much lower Michaelis constant than that of MAPKAPK1 isoforms) and MAPKAPK1 that are activated by ERK1 and ERK2 is required for full activation of transcription factors encoded by immediate-early genes, such as Fos and JunB, but not early growth response protein EGR1, another immediate-early gene product [622].
References
1.
Ezkurdia I, Del Pozo A, Frankish A, Rodriguez JM, Harrow J, Ashman K, Valencia A, Tress ML (2012) Comparative proteomics reveals a significant bias toward alternative protein isoforms with conserved structure and function. Molecular Biology and Evolution(mbe.oxfordjournals.org/content/early/2012/03/22/molbev.mss100.abstract)
2.
Chavent G (2010) Nonlinear Least Squares for Inverse Problems, Theoretical Foundations and Step-by-Step Guide for Applications. Springer, New York
3.
Bensoussan A (1971) Filtrage optimal des systèmes linéaires [Optimal filtering of linear systems] Dunod, Paris
4.
Bertoglio C, Moireau P, Gerbeau JF (2012) Sequential parameter estimation for fluid-structure problems. Application to hemodynamics. International Journal for Numerical Methods in Biomedical Engineering 28:434–455MathSciNet
5.
Lombardi D, Iollo A, Colin T, Saut O (2009) Inverse problems in tumor growth modelling (communication at CEMRACS summer school)
6.
Lagaert JB (2011) Modélisation de la croissance tumorale [Tumor Growth Modeling], PhD thesis, Bordeaux University
7.
van Meer G, Voelker DR, Feigenson GW (2008) Membrane lipids: where they are and how they behave. Nature Reviews – Molecular Cell Biology 9:112–124
8.
D’Arrigo P, Servi S (2010) Synthesis of lysophospholipids. Molecules 15:1354–1377
9.
Voet D, Voet JG (2011) Signal Transduction (Chap 19). Biochemistry (4th edition), Wiley, Hoboken, New Jersey
10.
Berridge MJ (2009) Module 2: Cell Signalling Pathways. Cell Signalling Biology. Biochemical Journal’s Signal Knowledge Environment Portland Press Ltd., London, UK (www.biochemj.org/csb/002/csb002.pdf)
11.
Delon C, Manifava M, Wood E, Thompson D, Krugmann S, Pyne S, Ktistakis NT (2004) Sphingosine kinase 1 is an intracellular effector of phosphatidic acid. Journal of Biological Chemistry 279:44763–44774
12.
Carricaburu V, Lamia KA, Lo E, Favereaux L, Payrastre B, Cantley LC, Rameh LE (2003) The phosphatidylinositol (PI)-5-phosphate 4-kinase type II enzyme controls insulin signaling by regulating PI-3,4,5-trisphosphate degradation. Proceedings of the National Academy of Sciences of the United States of America 100:9867–9872
13.
Maag D, Maxwell MJ, Hardesty DA, Boucher KL, Choudhari N, Hanno AG, Ma JF, Snowman AS, Pietropaoli JW, Xu R, Storm PB, Saiardi A, Snyder SH, Resnick AC (2011) Inositol polyphosphate multikinase is a physiologic PI3-kinase that activates Akt/PKB. Proceedings of the National Academy of Sciences of the United States of America 108: 1391–1396
14.
Weirich CS, Erzberger JP, Flick JS, Berger JM, Thorner J, Weis K (2006) Activation of the DExD/H-box protein Dbp5 by the nuclear-pore protein Gle1 and its coactivator InsP6 is required for mRNA export. Nature – Cell Biology 8:668–676
15.
Alcázar-Román AR, Tran EJ, Guo S, Wente SR (2006) Inositol hexakisphosphate and Gle1 activate the DEAD-box protein Dbp5 for nuclear mRNA export. Nature – Cell Biology 8: 711–716
16.
Macbeth MR, Schubert HL, VanDemark AP, Lingam AT, Hill CP, Bass BL (2005) Inositol hexakisphosphate is bound in the ADAR2 core and required for RNA editing. Science 309:1534–1539
17.
Huang YH, Grasis JA, Miller AT, Xu R, Soonthornvacharin S, Andreotti AH, Tsoukas CD, Cooke MP, Sauer K (2007) Positive regulation of Itk PH domain function by soluble IP4. Science 316:886–889
18.
Shen X, Xiao H, Ranallo R, Wu WH, Wudagger C (2003) Modulation of ATP-dependent chromatin-remodeling complexes by inositol polyphosphates. Science 299:112–114
19.
Mulugu S, Bai W, Fridy PC, Bastidas RJ, Otto JC, Dollins DE, Haystead TA, Ribeiro AA, York JD (2007) A conserved family of enzymes that phosphorylate inositol hexakisphosphate. Science 316:106–109
20.
Lee YS, Mulugu S, York JD, O’Shea EK (2007) Regulation of a cyclin-CDK-CDK inhibitor complex by inositol pyrophosphates. Science 316:109–112
21.
Saiardi A, Bhandari R, Resnick AC, Snowman AM, Snyder SH (2004) Phosphorylation of proteins by inositol pyrophosphates. Science 306:2101–2105
22.
Chakraborty A, Koldobskiy MA, Sixt KM, Juluri KR, Mustafa AK, Snowman AM, van Rossum DB, Patterson RL, Snyder SH (2008) HSP90 regulates cell survival via inositol hexakisphosphate kinase-2. Proceedings of the National Academy of Sciences of the United States of America 105:1134-1139
23.
Szado T, Vanderheyden V, Parys JB, De Smedt H, Rietdorf K, Kotelevets L, Chastre E, Khan F, Landegren U, Söderberg O, Bootman MD, Roderick HL (2008) Phosphorylation of inositol 1,4,5-trisphosphate receptors by protein kinase B/Akt inhibits Ca2+ release and apoptosis. Proceedings of the National Academy of Sciences of the United States of America 105: 2427–2432
24.
Otto JC, Kelly P, Chiou ST, York JD (2007) Alterations in an inositol phosphate code through synergistic activation of a G protein and inositol phosphate kinases. Proceedings of the National Academy of Sciences of the United States of America 104:15653–15658
25.
Zhang C, Majerus PW, Wilson MP (2012) Regulation of inositol 1,3,4-trisphosphate 5/6-kinase (ITPK1) by reversible lysine acetylation. Proceedings of the National Academy of Sciences of the United States of America 109:2290–2295
26.
Ohnishi T, Ohba H, Seo KC, Im J, Sato Y, Iwayama Y, Furuichi T, Chung SK, Yoshikawa T (2007) Spatial expression patterns and biochemical properties distinguish a second myo-inositol monophosphatase IMPA2 from IMPA1. Journal of Biological Chemistry 282: 637–646
27.
Berggard T, Szczepankiewicz O, Thulin E, Linse S (2002) Myo-inositol monophosphatase is an activated target of calbindin D28k. Journal of Biological Chemistry 277:41954–41959
28.
Irvine RF (2002) Nuclear lipid signaling. Science Signaling 150:re13
29.
Schouten A, Agianian B, Westerman J, Kroon J, Wirtz KWA, Gros P (2002) Structure of apo-phosphatidylinositol transfer protein α provides insight into membrane association. EMBO Journal 21:2117–2121
30.
Woodcock EA, Kistler PM, Ju YK (2009) Phosphoinositide signalling and cardiac arrhythmias. Cardiovascular Research 82:286–295
31.
Jao CY, Roth M, Welti R, Salic A (2009) Metabolic labeling and direct imaging of choline phospholipids in vivo. Proceedings of the National Academy of Sciences of the United States of America 106:15332–15337
32.
Breslow DK, Collins SR, Bodenmiller B, Aebersold R, Simons K, Shevchenko A, Ejsing CS, Weissman JS (2010) Orm family proteins mediate sphingolipid homeostasis. Nature 463:1048–1053
33.
Pavoine C, Pecker F (2009) Sphingomyelinases: their regulation and roles in cardiovascular pathophysiology. Cardiovascular Research 82:175–183
34.
Cogolludo A, Moreno L, Frazziano G, Moral-Sanz J, Menendez C, Castañeda J, González C, Villamor E, Perez-Vizcaino F (2009) Activation of neutral sphingomyelinase is involved in acute hypoxic pulmonary vasoconstriction. Cardiovascular Research 82:296–302
35.
Karliner JS (2009) Sphingosine kinase regulation and cardioprotection. Cardiovascular Research 82:184–192
36.
Means CK, Brown JH (2009) Sphingosine-1-phosphate receptor signalling in the heart. Cardiovascular Research 82:193–200
37.
Gellings Lowe N, Swaney JS, Moreno KM, Sabbadini RA (2009) Sphingosine-1-phosphate and sphingosine kinase are critical for transforming growth factor-beta-stimulated collagen production by cardiac fibroblasts. Cardiovascular Research 82:303–312
38.
Sanchez T, Skoura A, Wu MT, Casserly B, Harrington EO, Hla T (2007) Induction of vascular permeability by the sphingosine 1-phosphate receptor-2 (S1P2R) and its downstream effectors ROCK and PTEN. Arteriosclerosis, Thrombosis, and Vascular Biology 27:1312–1318
39.
Sattler K, Levkau B (2009) Sphingosine-1-phosphate as a mediator of high-density lipoprotein effects in cardiovascular protection. Cardiovascular Research 82:201–211
40.
Igarashi J, Michel T (2009) Sphingosine-1-phosphate and modulation of vascular tone. Cardiovascular Research 82:212-220
41.
Frias MA, James RW, Gerber-Wicht C, Lang U (2009) Native and reconstituted HDL activate Stat3 in ventricular cardiomyocytes via ERK1/2: Role of sphingosine-1-phosphate. Cardiovascular Research 82:313–323
42.
Ke Y, Lei M, Solaro RJ (2008) Regulation of cardiac excitation and contraction by p21 activated kinase-1. Progress in Biophysics and Molecular Biology 98:238–250
43.
Jenkins CM, Cedars A, Gross RW (2009) Eicosanoid signalling pathways in the heart. Cardiovascular Research 82:240–249
44.
Zhao J, O’Donnell VB, Balzar S, St Croix CM, Trudeau JB, Wenzel SE (2011) 15-Lipoxygenase 1 interacts with phosphatidylethanolamine-binding protein to regulate MAPK signaling in human airway epithelial cells. Proceedings of the National Academy of Sciences of the United States of America 108:14246–14251
45.
Jacobs ER, Zeldin DC (2001) The lung HETEs (and EETs) up. American Journal of Physiology – Heart and Circulatory Physiology 280:H1–H10
46.
Watanabe K (2011) Recent reports about enzymes related to the synthesis of prostaglandin (PG) F2 (PGF2α and 9α, 11β-PGF2). Journal of Biochemistry 150:593–596
47.
Yeaman SJ (2004) Hormone-sensitive lipase – new roles for an old enzyme. Biochemical Journal 379:11–22
48.
Okazaki H, Igarashi M, Nishi M, Sekiya M, Tajima M, Takase S, Takanashi M, Ohta K, Tamura Y, Okazaki S, Yahagi N, Ohashi K, Amemiya-Kudo M, Nakagawa Y, Nagai R, Kadowaki T, Osuga J, Ishibashi S (2008) Identification of neutral cholesterol ester hydrolase, a key enzyme removing cholesterol from macrophages. Journal of Biological Chemistry 283:33357–33364
49.
Jaye M, Lynch KJ, Krawiec J, Marchadier D, Maugeais C, Doan K, South V, Amin D, Perrone M, Rader DJ (1999) A novel endothelial-derived lipase that modulates HDL metabolism. Nature – Genetics 21:424–428
50.
Strauss JG, Zimmermann R, Hrzenjak A, Zhou Y, Kratky D, Levak-Frank S, Kostner GM, Zechner R, Frank S (2002) Endothelial cell-derived lipase mediates uptake and binding of high-density lipoprotein (HDL) particles and the selective uptake of HDL-associated cholesterol esters independent of its enzymic activity. Biochemical Journal 368:69–79
51.
Kojima Y, Ishida T, Sun L, Yasuda T, Toh R, Rikitake Y, Fukuda A, Kume N, Koshiyama H, Taniguchi A, Hirata KI (2010) Pitavastatin decreases the expression of endothelial lipase both in vitro and in vivo. Cardiovascular Research 87:385–393
52.
Yano M, Matsumura T, Senokuchi T, Ishii N, Murata Y, Taketa K, Motoshima H, Taguchi T, Sonoda K, Kukidome D, Takuwa Y, Kawada T, Brownlee M, Nishikawa T, Araki E (2007) Statins activate peroxisome proliferator-activated receptor γthrough extracellular signal-regulated kinase 1/2 and p38 mitogen-activated protein kinase-dependent cyclooxygenase-2 expression in macrophages. Circulation Research 100:1442–1451
53.
Favari E, Zanotti I, Zimetti F, Ronda N, Bernini F, Rothblat GH (2004) Probucol inhibits ABCA1-mediated cellular lipid efflux. Arteriosclerosis, Thrombosis, and Vascular Biology 24:2345–2350
54.
Aoki J, Inoue A, Makide K, Saiki N, Arai H (2007) Structure and function of extracellular phospholipase A1 belonging to the pancreatic lipase gene family. Biochimie 89:197–204
55.
Aoki J, Nagai Y, Hosono H, Inoue K, Arai H (2002) Structure and function of phosphatidylserine-specific phospholipase A1. Biochimica et Biophysica Acta 1582:26–32
56.
Wassum KM, Ostlund SB, Maidment NT, Balleine BW (2009) Distinct opioid circuits determine the palatability and the desirability of rewarding events. Proceedings of the National Academy of Sciences of the United States of America 106:12512–12517
57.
D’Agostino D, Lowe ME (2004) Pancreatic lipase-related protein 2 is the major colipase-dependent pancreatic lipase in suckling mice. Journal of Nutrition 134:132–134
58.
Alexander SPH, Mathie A, Peters JA (2009) Guide to Receptors and Channels (GRAC), 4th edn., British Journal of Pharmacology 158:S1–S254 (www3.interscience.wiley.com/ journal/122684220/issue)
59.
Burke JE, Dennis EA (2009) Phospholipase A2 structure/function, mechanism, and signaling. Journal of Lipid Research 50:S237–S242
60.
Schaloske RH, Dennis EA (2006) The phospholipase A2 superfamily and its group numbering system. Biochimica et Biophysica Acta (BBA) – Molecular and Cell Biology of Lipids 1761:1246–1259
61.
Rosa AO, Rapoport SI (2009) Intracellular- and extracellular-derived Ca2 + influence phospholipase A2-mediated fatty acid release from brain phospholipids. Biochimica et Biophysica Acta 1791:697–705
62.
Murakami M, Taketomi Y, Sato H, Yamamoto K (2011) Secreted phospholipase-A2 revisited. Journal of Biochemistry 150:233–255
63.
Lee JC, Simonyi A, Sun AY, Sun GY (2011) Phospholipases A2 and neural membrane dynamics: implications for Alzheimer’s disease. Journal of Neurochemistry 116:813–819
64.
Perrin-Cocon L, Agaugué S, Coutant F, Masurel A, Bezzine S, Lambeau G, André P, Lotteau V (2004) Secretory phospholipase A2 induces dendritic cell maturation. European Journal of Immunology 34:2293–2302
65.
Ancian P, Lambeau G, Mattéi MG, Lazdunski M (1995) The human 180-kDa receptor for secretory phospholipases A2. Molecular cloning, identification of a secreted soluble form, expression, and chromosomal localization. Journal of Biological Chemistry 270:8963–8970
66.
Triggiani M, Granata F, Oriente A, De Marino V, Gentile M, Calabrese C, Palumbo C, Marone G (2000) Secretory phospholipase A2 induce β-glucuronidase release and IL-6 production from human lung macrophages. Journal of Immunology 164:4908–4915
67.
Tada K, Murakami M, Kambe T, Kudo I (1998) Induction of cyclooxygenase-2 by secretory phospholipases A2 in nerve growth factor-stimulated rat serosal mast cells is facilitated by interaction with fibroblasts and mediated by a mechanism independent of their enzymatic functions. Journal of Immunology 161:5008–5015
68.
Ibeas E, Fuentes L, Martin R, Hernandez M, Nieto ML (2009) Secreted phospholipase A2 type IIA as a mediator connecting innate and adaptive immunity: new role in atherosclerosis. Cardiovascular Research 81(1):54-63
69.
Murakami M, Kudo I (2003) New phospholipase A2 isozymes with a potential role in atherosclerosis. Current Opinion in Lipidology 14:431–436
70.
Kuksis A, Pruzanski W (2008) Phase composition of lipoprotein SM/cholesterol/PtdCho affects FA specificity of sPLA2s. Journal of Lipid Research 49:2161–2168
71.
The HUGO Gene Nomenclature Committee (HGNC; www.genenames.org/genefamilies/ PNPLA) Phospholipases
72.
Wilson PA, Gardner SD, Lambie NM, Commans SA, Crowther DJ (2006) Characterization of the human patatin-like phospholipase family. Journal of Lipid Research 47:1940–1949
73.
Kienesberger PC, Oberer M, Lass A, Zechner R (2009) Mammalian patatin domain containing proteins: a family with diverse lipolytic activities involved in multiple biological functions. Journal of Lipid Research 50:S63–S68
74.
Sun GY, Shelat PB, Jensen MB, He Y, Sun AY, Simonyi A (2010) Phospholipases A2 and inflammatory responses in the central nervous system. Neuromolecular Medicine 12:133–148
75.
Ohto T, Uozumi N, Hirabayashi T, Shimizu T (2005) Identification of novel cytosolic phospholipase A2s, murine cPLA2δ, ε, and ζ, which form a gene cluster with cPLA2β. Journal of Biological Chemistry 280:24576–24583
76.
Hoffmann R, Valencia A (2004) A gene network for navigating the literature. Nature – Genetics 36:664 (Information Hyperlinked over Proteins www.ihop-net.org/)
77.
Alberghina M (2010) Phospholipase-A2: new lessons from endothelial cells. Microvascular Research 80:280–285
78.
Suh PG, Park JI, Manzoli L, Cocco L, Peak JC, Katan M, Fukami K, Kataoka T, Yun S, Ryu SH (2008) Multiple roles of phosphoinositide-specific phospholipase C isozymes. BMB Reports 41:415–434
79.
Waldo GL, Ricks TK, Hicks SN, Cheever ML, Kawano T, Tsuboi K, Wang X, Montell C, Kozasa T, Sondek J, Harden TK (2010) Kinetic scaffolding mediated by a phospholipase C-β and Gq signaling complex. Science 330:974–980
80.
Gutman O, Walliser C, Piechulek T, Gierschik P, Henis YI (2010) Differential regulation of phospholipase C-β2 activity and membrane interaction by Gαq, Gβ1γ2, and Rac2. Journal of Biological Chemistry 285:3905–3915
81.
Hunter I, Mascall KS, Ramos JW, Nixon GF (2011) A phospholipase Cγ1-activated pathway regulates transcription in human vascular smooth muscle cells. Cardiovascular Research 90:557–564
82.
von Essen MR, Kongsbak M, Schjerling P, Olgaard K, Odum N, Geisler C (2010) Vitamin D controls T cell antigen receptor signaling and activation of human T cells. Nature – Immunology 11:344–349
83.
Kobayashi M, Lomasney JW (2010) Phospholipase C δ4. UCSD-Nature Molecule Pages, UCSD-Nature Signaling Gateway (www.signaling-gateway.org)
84.
Wing MR, Bourdon DM, Harden TK (2003) PLC-ε: a shared effector protein in Ras-, Rho-, and Gαβγ-mediated signaling. Molecular Interventions 3:273–280
85.
Hu L, Edamatsu H, Takenaka N, Ikuta S, Kataoka T (2010) Crucial role of phospholipase Cepsilon in induction of local skin inflammatory reactions in the elicitation stage of allergic contact hypersensitivity. Journal of Immunology 184:993–1002
86.
Stewart AJ, Morgan K, Farquharson C, Millar RP (2007) Phospholipase C-η enzymes as putative protein kinase C and Ca2 + signalling components in neuronal and neuroendocrine tissues. Neuroendocrinology 86:243–248
87.
Nomikos M, Blayney LM, Larman MG, Campbell K, Rossbach A, Saunders CM, Swann K, Lai FA (2005) Role of phospholipase C-ζdomains in Ca2 + -dependent phosphatidylinositol 4,5-bisphosphate hydrolysis and cytoplasmic Ca2 + oscillations. Journal of Biological Chemistry 280:31011–31018
88.
Martinson EA, Scheible S, Greinacher A, Presek P (1995) Platelet phospholipase D is activated by protein kinase C via an integrin α2b β3-independent mechanism. Biochemical Journal 310:623–628
89.
Gironcel D, Racaud-Sultan C, Payrastre B, Haricot M, Borchert G, Kieffer N, Breton M, Chap H (1996) α2b β3-integrin mediated adhesion of human platelets to a fibrinogen matrix triggers phospholipase C activation and phosphatidylinositol 3’,4’-biphosphate accumulation. FEBS Letters 389:253–256
90.
Elvers M, Stegner D, Hagedorn I, Kleinschnitz C, Braun A, Kuijpers ME, Boesl M, Chen Q, Heemskerk JW, Stoll G, Frohman MA, Nieswandt B (2010) Impaired α2b β3 integrin activation and shear-dependent thrombus formation in mice lacking phospholipase D1. Science Signaling 3:ra1
91.
Mahankali M, Peng HJ, Henkels KM, Dinauer MC, Gomez-Cambronero J (2011) Phospholipase D2 (PLD2) is a guanine nucleotide exchange factor (GEF) for the GTPase Rac2. Proceedings of the National Academy of Sciences of the United States of America 108:19617–19622
92.
Medina-Tato DA, Ward SG, Watson ML (2007) Phosphoinositide 3-kinase signalling in lung disease: leucocytes and beyond. Immunology 121:448–461
93.
Foster FM, Traer CJ, Abraham SM, Fry MJ (2003) The phosphoinositide (PI) 3-kinase family. Journal of Cell Science 116:3037–3040
94.
Vanhaesebroeck B, Guillermet-Guibert J, Graupera M, Bilange B (2010) The emerging mechanisms of isoform-specific PI3K signalling. Nature Reviews – Molecular Cell Biology 11:329–341
95.
Yuan TL, Choi HS, Matsui A, Benes C, Lifshits E, Luo J, Frangioni JV, Cantley LC (2008) Class 1A PI3K regulates vessel integrity during development and tumorigenesis. Proceedings of the National Academy of Sciences of the United States of America 105:9739–9744
96.
Foukas LC, Berenjeno IM, Gray A, Khwaja A, Vanhaesebroeck B (2010) Activity of any class IA PI3K isoform can sustain cell proliferation and survival. Proceedings of the National Academy of Sciences of the United States of America 107:11381–11386
97.
Kurig B, Shymanets A, Bohnacker T, Prajwal, Brock C, Ahmadian MR, Schaefer M, Gohla A, Harteneck C, Wymann MP, Jeanclos E, Nürnberg B (2009) Ras is an indispensable coregulator of the class IB phosphoinositide 3-kinase p87/p110γ. Proceedings of the National Academy of Sciences of the United States of America 106:20312–20317
98.
Shinohara M, Terada Y, Iwamatsu A, Shinohara A, Mochizuki N, Higuchi M, Gotoh Y, Ihara S, Nagata S, Itoh H, Fukui Y, Jessberger R (2002) SWAP-70 is a guanine-nucleotide-exchange factor that mediates signalling of membrane ruffling. Nature 416:759–763
99.
Ihara S, Oka T, Fukui Y (2006) Direct binding of SWAP-70 to non-muscle actin is required for membrane ruffling. Journal of Cell Science 119:500–507
100.
Donald S, Humby T, Fyfe I, Segonds-Pichon A, Walker SA, Andrews SR, Coadwell WJ, Emson P, Wilkinson LS, Welch HC (2008) P-Rex2 regulates Purkinje cell dendrite morphology and motor coordination. Proceedings of the National Academy of Sciences of the United States of America 105:4483–4488
101.
Oudit GY, Penninger JM (2009) Cardiac regulation by phosphoinositide 3-kinases and PTEN. Cardiovascular Research 82:250–260
102.
Foukas LC, Claret M, Pearce W, Okkenhaug K, Meek S, Peskett E, Sancho S, Smith AJ, Withers DJ, Vanhaesebroeck B (2006) Critical role for the p110α phosphoinositide-3-OH kinase in growth and metabolic regulation. Nature 441:366–370
103.
Knight ZA, Gonzalez B, Feldman ME, Zunder ER, Goldenberg DD, Williams O, Loewith R, Stokoe D, Balla A, Toth B, Balla T, Weiss WA, Williams RL, Shokat KM (2006) A pharmacological map of the PI3-K family defines a role for p110α in insulin signaling. Cell 125:647–649
104.
Jia S, Liu Z, Zhang S, Liu P, Zhang L, Lee SH, Zhang J, Signoretti S, Loda M, Roberts TM, Zhao JJ (2008) Essential roles of PI(3)K-p110β in cell growth, metabolism and tumorigenesis. Nature 454:776–779
105.
Kumar A, Fernadez-Capetillo O, Carrera AC (2010) Nuclear phosphoinositide 3-kinase β controls double-strand break DNA repair. Proceedings of the National Academy of Sciences of the United States of America 107:7491–7496
106.
Hawkins PT, Stephens LR (2007) PI3Kγ is a key regulator of inflammatory responses and cardiovascular homeostasis. Science 318:64–66
107.
Park SJ, Lee KS, Kim SR, Min KH, Moon H, Lee MH, Chung CR, Han HJ, Puri KD, Lee YC (2010) Phosphoinositide 3-kinase δ inhibitor suppresses IL-17 expression in a murine asthma model. European Respiratory Journal (doi:10.1183/09031936.00106609)
108.
Marwick JA, Caramori G, Casolari P, Mazzoni F, Kirkham PA, Adcock IM, Chung KF, Papi A (2010) A role for phosphoinositol 3-kinase δ in the impairment of glucocorticoid responsiveness in patients with chronic obstructive pulmonary disease. Journal of Allergy and Clinical Immunology 125:1146–1153
109.
Beltran L, Chaussade C, Vanhaesebroeck B, Cutillas PR (2011) Calpain interacts with class IA phosphoinositide 3-kinases regulating their stability and signaling activity. Proceedings of the National Academy of Sciences of the United States of America 108:16217–16222
110.
Miller S, Tavshanjian B, Oleksy A, Perisic O, Houseman BT, Shokat KM, Williams RL (2010) Shaping development of autophagy inhibitors with the structure of the lipid kinase Vps34. Science 327:1638–1642
111.
Matsunaga K, Saitoh T, Tabata K, Omori H, Satoh T, Kurotori N, Maejima I, Shirahama-Noda K, Ichimura T, Isobe T, Akira S, Noda T, Yoshimori T (2009) Two Beclin 1-binding proteins, Atg14L and Rubicon, reciprocally regulate autophagy at different stages. Nature – Cell Biology 11:385–396
112.
Yan Y, Flinn RJ, Wu H, Schnur RS, Backer JM (2009) hVps15, but not Ca2 + /CaM, is required for the activity and regulation of hVps34 in mammalian cells. Biochemical Journal 417: 747–755
113.
Okada M, Jang SW, Ye K (2008) Akt phosphorylation and nuclear phosphoinositide association mediate mRNA export and cell proliferation activities by ALY. Proceedings of the National Academy of Sciences of the United States of America 105:8649–8654
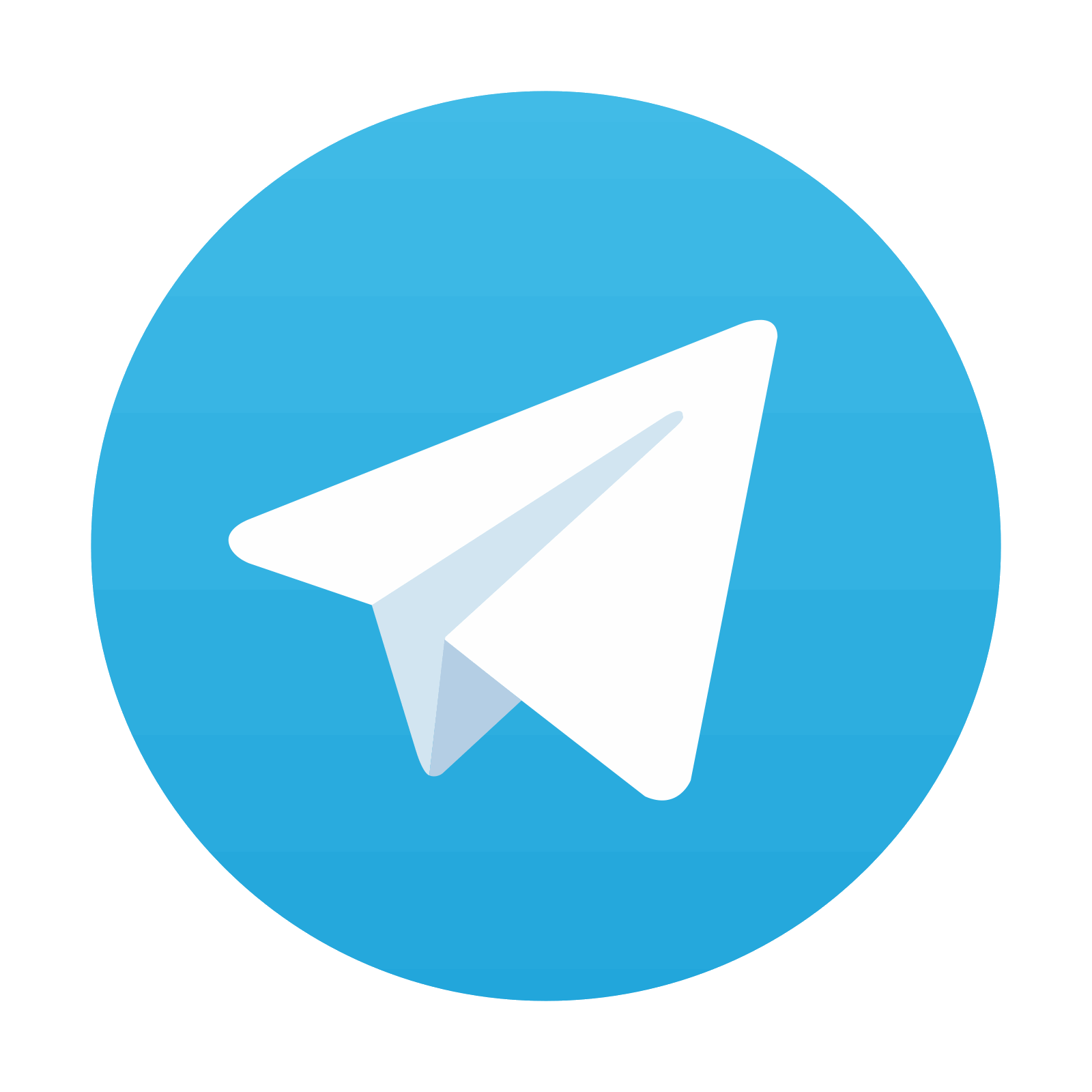
Stay updated, free articles. Join our Telegram channel
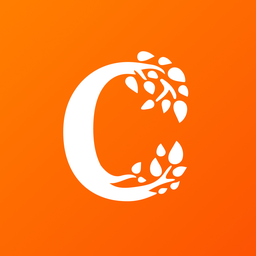
Full access? Get Clinical Tree
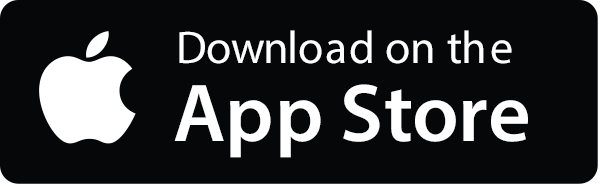
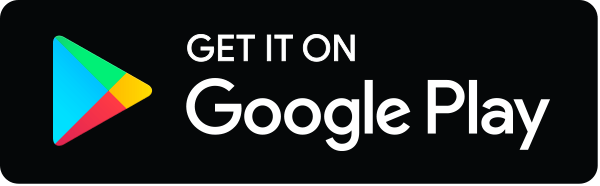
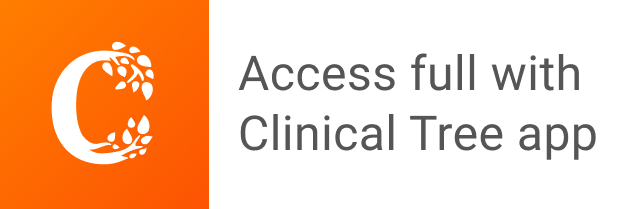