Fig. 9.1
How the main types of EVs are formed. Microvesicles are released from the plasma membrane via a process of shedding or budding. Vesicles form within early endosomes by invagination of the endosome membrane, resulting in what are called multivesicular bodies. Once the multivesicular body fuses with the plasma membrane, the exosomes within are released from the cell into the extracellular space
Most, if not all, cell types appear to be capable of releasing exosomes and microvesicles (Raposo and Stoorvogel 2013), but some are more prolific than others. Platelets are some of the most prolific, as discovered by Peter Wolf in 1967, when he realised that “platelet dust ” released by activated platelets was actually microvesicles. At the time, however, they were considered to be cellular debris (Wolf 1967). In addition to releasing microvesicles, platelets also produce abundant numbers of exosomes (Heijnen et al. 1999). Exosomes were first discovered by examining the particles released by reticulocytes during their maturation into red blood cells (Johnstone et al. 1987). Again, they were initially thought to be waste products involved in “trash disposal” of proteins such as the transferrin receptor that were no longer required (Johnstone et al. 1987). Dendritic cells were also found to release exosomes, but these were shown to have a functional effect, since by presenting major histocompatibility complex antigens and generating an immune response, allogeneic donor-derived exosomes could induce immune tolerance to a heart allograft (Peche et al. 2003). In 2001, tumour cells were shown to release large numbers of exosomes which, when taken up by dendritic cells, could initiate a cytotoxic T-cell response (Wolfers et al. 2001). Exosomes have remained an intensive area of research in the cancer field, although the general opinion is now that exosomes aid the tumour cells in escaping immune surveillance by suppressing the immune response (Zhang and Grizzle 2011), and may facilitate metastasis (Hood et al. 2011).
In the cardiovascular system, exosomes have been isolated from primary adult rat cardiomyocytes (Gupta and Knowlton 2007; Wang et al. 2014) as well as the HL-1 mouse atrial cardiomyocyte cell line (Genneback et al. 2013; Waldenstrom et al. 2012). Matrix vesicles , which are secreted by vascular smooth muscle cells and are involved in initiating vascular calcification, were recently shown to be exosomes (Kapustin et al. 2015). Endothelial cells appear to release both exosomes and microvesicles (Deregibus et al. 2007). The production of exosomes is difficult to confirm in vivo. Vesicle-like structures resembling exosomes have been identified in electron micrographs of mouse heart (Barile et al. 2012), but in order to confirm their identity as exosomes and not unrelated vesicles or cross-sections of finger-like projections, for example, it would be necessary to immunolabel them with antibodies against known exosomal markers proteins . It is also very difficult to determine cell of origin in static images. Vascular smooth muscle cells secrete matrix vesicles that initiate vascular calcification . These vesicles were recently identified as exosomes containing some proteins to osteoblast-derived matrix vesicles (Kapustin et al. 2015), demonstrating an intriguing role for exosomes in vascular pathology. Finally, all body fluids contain large numbers of exosomes and microvesicles (Yellon and Davidson 2014; Thery et al. 2006). In the case of blood, the majority of EVs originate from platelets (Smalley et al. 2007), though erythrocytes, endothelium, lymphocytes or other blood cells also contribute.
9.3 Isolation of Extracellular Vesicles
In all studies of EVs the isolation method used is a primary consideration. This is particularly the case for exosomes, for which there is as yet no “perfect” method of purification. In fact, the International Society of Extracellular Vesicles (ISEV) released a position statement in 2014 recognizing that most isolation methods result in a mixture of different types of EVs (Lotvall et al. 2014). This is partly a consequence of the functional definition of exosomes as “vesicles that are released from multivesicular bodies”—once released from the cell there is no unique method to identify their origin. It is also experimentally challenging to use size to separate exosomes from microvesicles, which are only slightly larger, and from other vesicles, protein aggregates, and lipoproteins, which can overlap in size (Lotvall et al. 2014; Thery et al. 2006). Density gradient centrifugation is often used to separate different vesicle types based on their buoyant density (Lotvall et al. 2014; Thery et al. 2006). This can be useful for separating them from protein aggregates which do not float, but does not completely eliminate lipoproteins (HDL and LDL ) (Lotvall et al. 2014). Protein markers are commonly used to identify exosomes and microvesicles in preparations, and these are discussed under the following section. In general, cardiovascular researchers will be interested in isolating exosomes either from in vitro cultured cells , or from blood. The critical issues for each are somewhat different and are addressed separately.
With regard to the isolation of vesicles from in vitro cultured cells, most cell lines are grown in the presence of serum, which contains enormous quantities of exosomes. It is therefore essential to obtain specially filtered, certified exosome-free serum, or to prepare one’s own by overnight ultracentrifugation of the serum at 100,000 g or higher. Alternatively, it may be possible to grow some cell lines in the absence of serum during the experiment, although care must be taken that the cells remain healthy and do not undergo apoptosis, which will cause the release of apoptotic bodies that can co-purify with microvesicles or exosomes. The most efficient way of collecting exosomes from large volumes of cell medium is serial centrifugation. Sometimes, a filtration step is used first to concentrate the exosomes, however this is prone to artifactual generation of exosome-sized vesicles by fragmentation of the larger vesicles (Lotvall et al. 2014). In the process of serial centrifugation, the supernatant is collected and damaged cells and larger vesicles are removed by centrifugation at 10,000 for 30 min. The supernatant is then ultracentrifuged, typically at 100,000 g for 60 min to pellet exosomes (Hergenreider et al. 2012; Thery et al. 2006). As mentioned, the isolated exosome-rich fraction may be further purified by density gradient centrifugation on sucrose or iodixanol to enrich exosomes which have a density of between 1.13 and 1.19 g/mL (Yuana et al. 2014; Thery et al. 2006). This fraction is then diluted in buffer and re-centrifuged to remove the sucrose or iodixanol. One alternative method is to separate exosomes by size-exclusion chromatography (Boing et al. 2014). Although effective, this is less widely used due to lower throughput. A further technique is to use antibody affinity capture on beads or columns, although this requires that one is certain of the antigen marking the vesicles of interest, and the yield is much lower. Several companies now market reagents that can be used to rapidly precipitate exosomes from culture medium (or serum), the original being “Exoquick™ ”. Although yield is high, the purity is suspect (Van Deun et al. 2014), since it is difficult to wash the isolated exosome pellet washed without resorting to ultracentrifugation.
The isolation of EVs from blood is further complicated by pre-analytical considerations (Yuana et al. 2011). These are mostly designed to prevent or minimize the activation of platelets , which will otherwise release enormous numbers of microvesicles and exosomes which may influence the results. The clotting step involved in serum preparation causes massive platelet activation, which can be useful if large numbers of platelet vesicles are desired. However it is necessary to use plasma to obtain a selection of EVs representative of blood EVs (Yuana et al. 2011). Best practise is to use a relatedly large bore butterfly needle and draw blood into citrated Vacutainer® tubes, discarding the first tube. After mixing gently by inversion, the tube is centrifuged for at 1600×g for 20 min at room temperature (to minimize platelet activation) and the platelet-free plasma is carefully collected from the top. The standard protocol (Thery et al. 2006) is then to centrifuge the plasma at 10,000×g for 30 min at room temperature to pellet microvesicles. The supernatant containing exosomes is removed and ultracentrifuged at 100,000×g for 90 min at 4 °C. The supernatant is removed and the pellet resuspended in Ca2+ and Mg2+ free PBS containing citrate, and ultracentrifuged again. After a final wash, the pellet (which is not usually visible) can be vigorously resuspended for analysis of exosomes. Similar to exosomes isolated from tissue culture, density gradient purification may be performed to improve purity from protein aggregates, although contamination with high-density lipoproteins is very difficult to eliminate (Yuana et al. 2014).
Although some publications have used precipitation reagents such as Exoquick™ to isolate exosomes from blood, the standard protocol was designed for use with serum and must be adapted by the addition of Thromboplastin D in order to remove coagulative proteins and enable the use of plasma. Furthermore, the difficulty of washing the pellet containing exosomes is a major issue, and since blood contains very high concentrations of proteins, microvesicles, etc. some of these will remain in the final isolate. Despite these issues, some researchers have successfully used these methods to identify signature differences in exosome content between groups of individuals, which may be useful for diagnostic purposes. However, it must be taken into consideration that the exosomal origin of these samples remains unproven.
A promising new technique combining the convenience of precipitation and a pre-made kit with the improved separation of columns is the Exo-Spin™ kit , which appear to provide a convenient and reproducible means of eliminating >95 % of non-vesicular protein from biological fluid samples such as plasma (Welton et al. 2015).
9.4 Characterization of Exosomes and Microvesicles
Several approaches are necessary to unambiguously identify exosomes. Since their diameter is smaller than the wavelength of light, direct imaging of individual exosome using standard microscopy is impossible. Furthermore, they lie well below the detection threshold of flow cytometry (Nolte-’t Hoen et al. 2012). Flow cytometry is feasible for analysis of microvesicles but specialized modifications to flow cytometer equipment are necessary to optimize detection of particles at the lower range of size (Nolte-’t Hoen et al. 2012).
The most direct means of visualizing EVs is by transmission electron micrography after drying and uranyl acetate staining (Thery et al. 2006). Exosomes and other vesicles exhibit a shape that has been called “cup-shaped ” as the vesicles collapse when dried (Fig. 9.2a) (Thery et al. 2006; Raposo and Stoorvogel 2013). Contaminating objects can include lipoproteins (which appear spherical), protein aggregates, cellular organelles or viruses. Sometimes the background of the electron micrograph grid itself can resemble objects of the appropriate size range for exosomes, so it is useful to include a control grid without exosomes for comparison. Positive identification by electron micrograph is an important step in confirming the presence of exosomes and calculating the size distribution of a vesicle population.
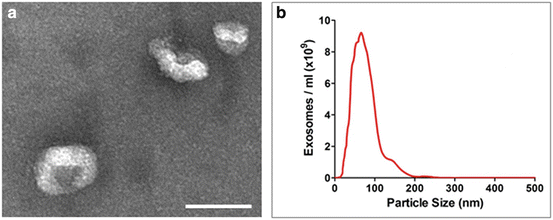
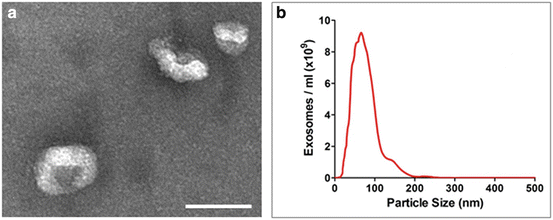
Fig. 9.2
(a) Exosomes isolated from human plasma by serial centrifugation and visualized by electron micrography. Scale = 100 nm. (b) Size distribution of human plasma exosomes determined by nanoparticle tracking analysis. The majority are 50–100 nm although some larger particles are also present
Vesicle size can also be determined using dynamic light scattering (DLS) or nanoparticle tracking analysis (NTA) (Fig. 9.2b) (Dragovic et al. 2011). DLS can be effective but when a vesicle preparation contains populations of different sizes NTA is more appropriate as it is able to measure the size of individual particles.
Exosomes contain particular protein markers as a consequence of their release mechanism from cells, and these are often used to aid their identification (or utilized for vesicle purification by antibody affinity). Foremost amongst these proteins are the tetraspanins, particularly CD9, CD63, CD81, although it must be recognized that other vesicles can express these molecules as well (Kowal et al. 2014). It may be possible to detect these proteins by Western blot analysis, although non-reducing gels should be used because the tetraspanins are heavily glycosylated (Thery et al. 2006). If it is difficult to obtain sufficient protein for a Western blot, an effective alternative is to adhere the exosomes onto 4 μm diameter latex beads, incubate them with fluorescent antibodies against tetraspanins or other marker proteins, and measure the fluorescence of individual beads separated by flow cytometry (Thery et al. 2006). Modern flow cytometers are quite capable of analysing most microvesicles (Ayers et al. 2014), although the smallest microvesicles might be beyond the range of detection without careful optimization of the flow cytometer.
It is recommended to use several of the above approaches in order to confirm the identity and origin of the EVs.
9.5 Extracellular Vesicles Can Transfer Proteins and RNA
EVs have been demonstrated to possess a number of interesting capabilities including the ability to deliver proteins and RNA to cells. There is some evidence that this also occurs in vivo , despite this being much more difficult to prove. One of the first proteins demonstrated to be transferred to recipient cells in vitro was tissue factor (TF) . TF was released from platelets within microvesicles that were subsequently incorporated into the membranes of monocytes and other cells where TF exerts its biological effects (Del Conde et al. 2005; Scholz et al. 2002). An important paper demonstrated that microvesicles from tumour cells could transfer a truncated, oncogenic form of the epidermal growth factor receptor between cells, leading to activation of transforming signalling pathways (MAPK and Akt) and horizontal propagation of oncogenes and their associated transforming phenotype (Al-Nedawi et al. 2008).
Exosomes are usually found to contain primarily cytosolic and membrane-localized proteins (Mathivanan et al. 2010). Certain proteins are found almost universally in exosomes due to their being part of the packaging and/or release pathway. These include the proteins Alix, TSP101, HSP70 and tetraspanin family proteins CD9, CD63, CD81, etc., which are therefore often used as markers proteins to indicate the presence of exosomes, as described in the previous section. The degree of sorting of proteins into exosomes and microvesicles appears to be greater when the proteins contain domains that link them to the plasma membrane (Shen et al. 2011), presumably as this makes them more likely to be incorporated into EVs as the plasma membrane buds off or invaginates to produce multivesicular bodies . There is some evidence that the protein and RNA content of exosomes depends on the cellular state (de Jong et al. 2012).
Similar to microvesicles, exosomes can not only deliver signalling proteins to cells, but be taken up by other cells, incorporating the protein into the recipient membrane. For example, the Notch ligand Delta-like 4 (Dll4) is incorporated into endothelial exosomes which can transfer the Dll4 protein to other endothelial cells and incorporate it into their cell membrane, resulting in an inhibition of Notch signalling and altered angiogenesis (Sheldon et al. 2010). Of particular interest are the examples in which exosomes have been shown to deliver protective proteins to recipient cells. αB crystallin was shown to be secreted from human retinal pigment epithelium in exosomes, and taken up by adjacent photoreceptors in mouse retinal explants exposed to oxidative stress (Sreekumar et al. 2010). An important theoretical consideration is the extent to which transfer of a relatively small number of copies of a protein can be realistically expected to have a physiological effect in the recipient cell in vivo . In this respect, it is easier to imagine transfer of small quantities of mRNA or miRNA being capable of having more dramatic effects.
In a seminal paper, Valadi et al, were first to show that exosomes can transfer mRNA and miRNA between cells (Valadi et al. 2007). This study used mast cells to demonstrate the transfer of functional mRNAs that were subsequently translated in the recipient cells. In an important control experiment, exosomes were pre-treated with RNAse and trypsin, to demonstrate that mRNA was protected within the vesicles and not simply associated or co-purified. Tumour-derived microvesicles were also shown to transfer mRNA to recipient cells that could be translated (Skog et al. 2008).
The profile of miRNAs contained within exosomes appears to differ according to the cell type of origin. For example, C2C12 myoblasts and C2C12 differentiated into myotubes released exosomes with different miRNA profiles (Forterre et al. 2014). Interestingly, the miRNA profile was different from that of the donor cells, indicating that there is selective packaging of miRNA into exosomes (Forterre et al. 2014). The mechanism of selective sorting of miRNA into exosomes is only beginning to be unravelled, but is believed to involve recognition of particular sequence motifs by sumoylated heterogeneous nuclear ribonucleoprotein A2B1 (hnRNPA2B1) (Villarroya-Beltri et al. 2013). The exosomes secreted by C2C12 myotubes were functional, being able to suppress expression of Sirt1 when taken up by myoblasts, potentially modulating metabolic homeostasis and contributing to the commitment of myoblasts during differentiation (Forterre et al. 2014).
Cardiac fibroblasts secrete miRNA-enriched exosomes, including miR-21-3p—a passenger strand miRNA which normally undergoes intracellular degradation (Bang et al. 2014). However, when these exosomes were taken up by neonatal cardiomyocytes in co-culture, the cardiomyocytes increased in size indicating a hypertrophic response (Bang et al. 2014). Thus it appears that different cells in the heart may communicate to each other via exosomes. A further significant exchange of miRNAs between cells of the cardiovascular system was shown to occur between endothelial cells and smooth muscle cells . In this case, EVs were secreted by endothelial cells that had been stimulated by shear stress, which is known to be atheroprotective (Hergenreider et al. 2012). The EVs were taken up into smooth muscle cells in co-culture, delivering miR-143/145 and controlling the expression of target genes (Hergenreider et al. 2012). They also reduced atherosclerotic lesion formation in the aorta of ApoE(−/−) mice (Hergenreider et al. 2012). Since a maximum centrifugation speed of 20,500×g was used to pellet EVs, and the size range of most of the vesicles on electron micrographs ranged between 60 and 130 nm, they were referred to conservatively as “extracellular vesicles”, but presumably contained a mix of exosomes and microvesicles .
In view of the RNA content of EVs which is related to the cell type of origin, and can alter in pathological settings, they have become an attractive source of biomarkers for profiling and identification of disease markers (Cheng et al. 2014; Jansen et al. 2014), as has been reviewed elsewhere (Gaceb et al. 2014).
9.5.1 Extracellular Vesicles Can Transfer Proteins and RNA
EVs have been demonstrated to possess a number of interesting capabilities including the ability to deliver proteins and RNA to cells. There is some evidence that this also occurs in vivo, despite this being much more difficult to prove. One of the first proteins demonstrated to be transferred to recipient cells in vitro was tissue factor (TF). TF was released from platelets within microvesicles that were subsequently incorporated into the membranes of monocytes and other cells where TF exerts its biological effects (Del Conde et al. 2005; Scholz et al. 2002). An important paper demonstrated that microvesicles from tumour cells could transfer a truncated, oncogenic form of the epidermal growth factor receptor between cells, leading to activation of transforming signalling pathways (MAPK and Akt) and horizontal propagation of oncogenes and their associated transforming phenotype (Al-Nedawi et al. 2008).
Exosomes are usually found to contain primarily cytosolic and membrane-localized proteins (Mathivanan et al. 2010). Certain proteins are found almost universally in exosomes due to their being part of the packaging and/or release pathway. These include the proteins Alix, TSP101, HSP70 and tetraspanin family proteins CD9, CD63, CD81, etc., which are therefore often used as markers proteins to indicate the presence of exosomes, as described in the previous section. The degree of sorting of proteins into exosomes and microvesicles appears to be greater when the proteins contain domains that link them to the plasma membrane (Shen et al. 2011), presumably as this makes them more likely to be incorporated into EVs as the plasma membrane buds off or invaginates to produce multivesicular bodies. There is some evidence that the protein and RNA content of exosomes depends on the cellular state (de Jong et al. 2012).
Similar to microvesicles, exosomes can not only deliver signalling proteins to cells, but be taken up by other cells, incorporating the protein into the recipient membrane. For example, the Notch ligand Delta-like 4 (Dll4) is incorporated into endothelial exosomes which can transfer the Dll4 protein to other endothelial cells and incorporate it into their cell membrane, resulting in an inhibition of Notch signalling and altered angiogenesis (Sheldon et al. 2010). Of particular interest are the examples in which exosomes have been shown to deliver protective proteins to recipient cells. αB crystallin was shown to be secreted from human retinal pigment epithelium in exosomes, and taken up by adjacent photoreceptors in mouse retinal explants exposed to oxidative stress (Sreekumar et al. 2010). An important theoretical consideration is the extent to which transfer of a relatively small number of copies of a protein can be realistically expected to have a physiological effect in the recipient cell in vivo. In this respect, it is easier to imagine transfer of small quantities of mRNA or miRNA being capable of having more dramatic effects.
In a seminal paper, Valadi et al, were first to show that exosomes can transfer mRNA and miRNA between cells (Valadi et al. 2007). This study used mast cells to demonstrate the transfer of functional mRNAs that were subsequently translated in the recipient cells. In an important control experiment, exosomes were pre-treated with RNAse and trypsin, to demonstrate that mRNA was protected within the vesicles and not simply associated or co-purified. Tumour-derived microvesicles were also shown to transfer mRNA to recipient cells that could be translated (Skog et al. 2008).
The profile of miRNAs contained within exosomes appears to differ according to the cell type of origin. For example, C2C12 myoblasts and C2C12 differentiated into myotubes released exosomes with different miRNA profiles (Forterre et al. 2014). Interestingly, the miRNA profile was different from that of the donor cells, indicating that there is selective packaging of miRNA into exosomes (Forterre et al. 2014). The mechanism of selective sorting of miRNA into exosomes is only beginning to be unravelled, but is believed to involve recognition of particular sequence motifs by sumoylated heterogeneous nuclear ribonucleoprotein A2B1 (hnRNPA2B1) (Villarroya-Beltri et al. 2013). The exosomes secreted by C2C12 myotubes were functional, being able to suppress expression of Sirt1 when taken up by myoblasts, potentially modulating metabolic homeostasis and contributing to the commitment of myoblasts during differentiation (Forterre et al. 2014).
Cardiac fibroblasts secrete miRNA-enriched exosomes, including miR-21-3p—a passenger strand miRNA which normally undergoes intracellular degradation (Bang et al. 2014). However, when these exosomes were taken up by neonatal cardiomyocytes in co-culture, the cardiomyocytes increased in size indicating a hypertrophic response (Bang et al. 2014). Thus it appears that different cells in the heart may communicate to each other via exosomes. A further significant exchange of miRNAs between cells of the cardiovascular system was shown to occur between endothelial cells and smooth muscle cells. In this case, EVs were secreted by endothelial cells that had been stimulated by shear stress, which is known to be atheroprotective (Hergenreider et al. 2012). The EVs were taken up into smooth muscle cells in co-culture, delivering miR-143/145 and controlling the expression of target genes (Hergenreider et al. 2012). They also reduced atherosclerotic lesion formation in the aorta of ApoE(−/−) mice (Hergenreider et al. 2012). Since a maximum centrifugation speed of 20,500 g was used to pellet EVs, and the size range of most of the vesicles on electron micrographs ranged between 60 and 130 nm, they were referred to conservatively as “extracellular vesicles”, but presumably contained a mix of exosomes and microvesicles.
In view of the RNA content of EVs which is related to the cell type of origin, and can alter in pathological settings, they have become an attractive source of biomarkers for profiling and identification of disease markers (Cheng et al. 2014; Jansen et al. 2014), as has been reviewed elsewhere (Gaceb et al. 2014).
9.6 Exosomes as a Potential Therapy for Cardiovascular Disease
Coronary artery disease is a major challenge facing health care systems around the world. In 2004, ischaemic heart disease was responsible for 7.2 million deaths, making it the leading cause of death. Myocardial infarction is a major cause of mortality and morbidity in patients with coronary artery disease. Heart failure often develops subsequently, depending on the extent of myocardial infarction. Therefore, treatment modalities that protect the heart from injury can be expected to result in major improvements not only in early mortality but on-going morbidity. Currently early reperfusion (restoration of normal coronary flow) remains the mainstay of treatment for those suffering an acute myocardial infarction. However, reperfusion also causes injury to heart muscle in what is termed “lethal reperfusion injury ” (Hausenloy and Yellon 2013). Final infarct size in patients with ST elevation myocardial infarction predicts long-term clinical outcome (Lonborg et al. 2013). Therefore, finding ways to minimize reperfusion injury is one approach that would be expected to improve the overall, long-term management of patients presenting with myocardial infarction (Braunwald and Kloner 1985; Piper et al. 1998; Hausenloy and Yellon 2013). Another theoretical approach would be to replace the contractile cardiomyocytes that were lost, by the use of stem cell therapy. As discussed elsewhere in this book, however, the results of this approach have been largely disappointing. On the other hand, some improvements in cardiac function have been observed after stem cell therapy , even in the absence of new cardiomyocytes formation . Since some benefit could also be observed experimentally after injection of conditioned medium from stem cells, the “paracrine” hypothesis developed in which it is proposed that engrafted stem cells release cytokines, growth factors and other proteins which act on cardiomyocytes and other myocardial proteins to confer improvements in function. Stem cells release a host of different factors (“the secretome”), many of which are pro-survival and proangiogenic (Makridakis et al. 2013). While many of these are released via the usual protein secretion pathways, the possibility was raised that they might be released together associated with exosomes, enabling a co-ordinated, and perhaps directed response to multiple factors.
The first study to examine whether exosomes released from stem cells are cardioprotective was performed in 2010 using an acute model of myocardial infarction . Timmers et al. had previously showed that conditioned medium from human ESC-derived mesenchymal stem cells (ESC-MSC) reduced infarct size in isolated, perfused mouse hearts subject to ischaemia and reperfusion (Timmers et al. 2007). The same group then purified exosomes from the conditioned medium by HPLC size-exclusion fractionation , and confirmed that these exosomes were sufficient to confer cardioprotection both in vitro and in vivo (Lai et al. 2010a). Long term protection with improved function after 28 days was observed after injection of at least 4 μg/kg into mice via the tail vein, just before reperfusion (Arslan et al. 2013). Interestingly, it appears that the exosomes had to be intact to induce protection, since they were no longer protective after homogenization (Arslan et al. 2013), although whether exosomes are disrupted after homogenization is not clear and was not demonstrated. Exosome administration caused an increase in the activity of cardioprotective kinases Akt and GSK3α/β after 1 h, and these remained activated until the day following treatment (Arslan et al. 2013). These kinases are known to be highly cardioprotective (Hausenloy et al. 2005). Significantly, there was also a reduction in myocardial oxidative stress, and local and systemic inflammation (Arslan et al. 2013). Exosomes isolated from MSC cells overexpressing GATA4 have also been shown to restore cardiac contractile function and reduce infarct size when injected intramyocardially at the time of infarction in a rat model (Yu et al. 2014). An increase in miR-19a was detected in the treated hearts, although it is difficult to be certain that this originated from the MSC exosomes and was not a response to treatment with exosomes (Yu et al. 2014). In turn, levels of PTEN, a predicted target of miR-19a, were reduced and Akt and ERK activation was increased (Yu et al. 2014). As an alternative cell source for large-scale production of exosomes which does not require ES cells, MSC cultures were developed from limb, kidney and liver tissues of three first trimester aborted foetuses. Despite absence of pluripotency-associated markers, these MSC were also an excellent source of cardioprotective exosomes (Lai et al. 2010b). Of interest, the protective pathways activated by exosomes appear to be applicable to other organs, since MSC exosomes (from human umbilical cord) have also been shown to protect against cisplatin-induced renal oxidative stress and apoptosis via ERK (Zhou et al. 2013), and to promote functional recovery after stroke in rats (Xin et al. 2013). Furthermore, it may be that protection is not limited to exosomes, as microvesicles derived from human adult mesenchymal stem cells also protected against ischaemia and reperfusion kidney injury (Gatti et al. 2011).
MSC are not the only type of stem cell that have been shown to release exosomes with beneficial cardiovascular effects. Intramyocardial injection of cardiac progenitor cells (CPCs) isolated from adult hearts improved cardiac function in animal models of myocardial infarction (Messina et al. 2004; Smith et al. 2007), although paracrine effects were found to contribute to the majority of the effect (Chimenti et al. 2010). Subsequently, exosomes from murine CPCs were found to reduce apoptosis when injected intramyocardially during ischaemia (Chen et al. 2013). In this study, however, exosomes were isolated by PEG precipitation (Chen et al. 2013), and therefore the more appropriate control would have been injection with PEG alone. Another study used several alternative methods of isolation of CPC-EVs: Exoquick™ precipitation, ultracentrifugation, or size-exclusion, column purification (Barile et al. 2014). Although more of tetraspanin marker proteins CD63 and CD81 were measured using Exoquick™, the three techniques were not rigorously compared, and as mentioned above, the Exoquick™ technique has some drawbacks—particularly the inability to wash the pellet to remove the Exoquick™ solution from the exosomes. Injection of these CPCs-EVs into the hearts of rats subject to permanent coronary artery ligation reduced cardiomyocyte apoptosis and scar size, increased the amount of viable tissue in the infarct area, increased blood vessel density, and prevented the loss of impairment of ventricular function between day 2 and day 7 ((Barile et al. 2014). In contrast, exosomes isolated from normal human dermal fibroblasts exhibited no benefit (Barile et al. 2014). Gray et al. used ultracentrifugation to purify exosomes from CPCs, and found that the rate of secretion of exosomes was increased after 3–12 h hypoxia (Gray et al. 2015). Intramyocardial injection of 5 μg/kg of the exosomes produced after hypoxia improved cardiac function and reduced fibrosis 21 days after ischaemia and reperfusion injury in rats (Gray et al. 2015). Interestingly, however, there was no benefit with exosomes from normoxic CPCs (Barile et al. 2014). The exosomes released after hypoxia had an altered miRNA content, and co-regulated miRNA with a beneficial profile were identified (Gray et al. 2015). Although cardiac endothelial cells and fibroblasts took up fluorescently stained exosomes in vitro, uptake was minimal in primary rat cardiomyocytes (Gray et al. 2015), suggesting either that they deliver miRNA directly to the former cells types, or that they interact with surface receptors on cardiomyocytes without delivering miRNA intracellularly. Thus, the exact mechanism of functional benefit conferred by exosomes remains unclear.
< div class='tao-gold-member'>
Only gold members can continue reading. Log In or Register a > to continue
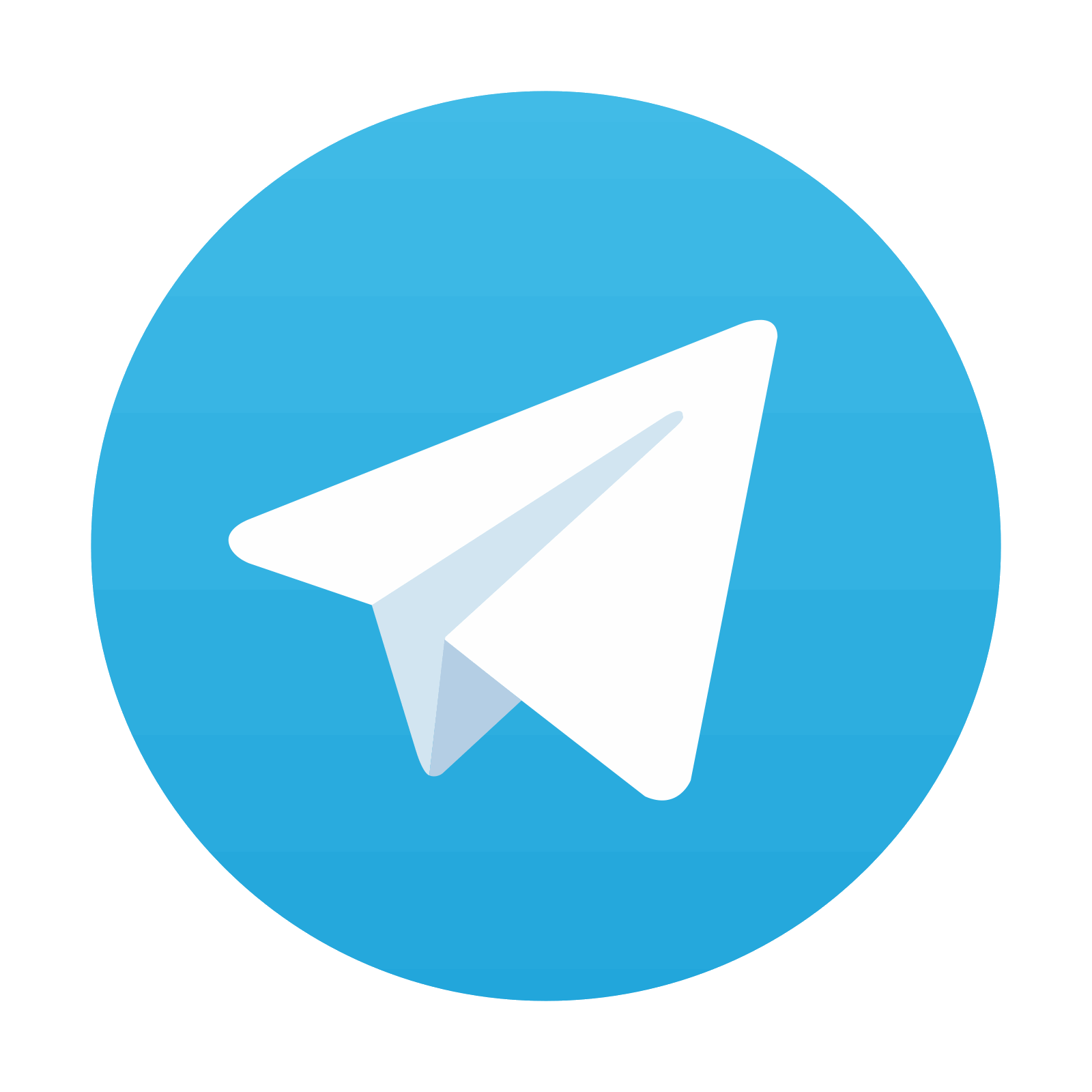
Stay updated, free articles. Join our Telegram channel
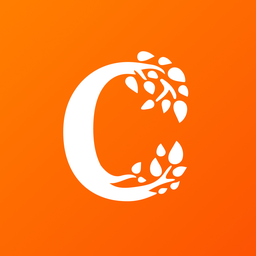
Full access? Get Clinical Tree
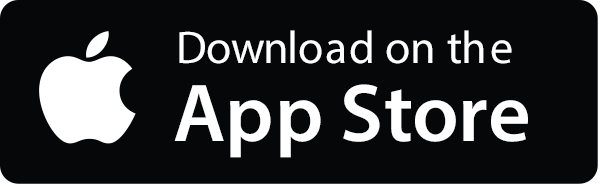
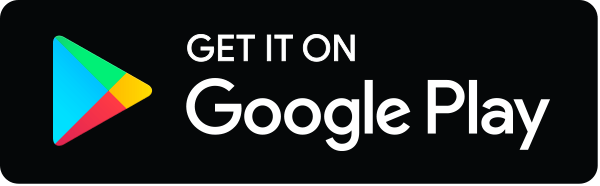