Key Points
- •
Cryodestruction occurs through two primary mechanisms: immediate and delayed injury.
- •
The use of low temperature as a thermal therapy provides a mode of transient injury that can be a useful tool in ablative procedures.
- •
Cryosurgical ablation produces a highly demarcated necrotic lesion approximating the frozen volume.
- •
Target tissue temperatures reaching at least −40°C are recommended to ensure cell ablation for tumors, but a broad range of thermal sensitivity at warmer freezing temperatures exists for non-neoplastic cells and tissues.
- •
The extent of tissue damage is related to the temperature-time exposure, particularly in the periphery of the frozen volume.
The underlying biological mechanisms in action during targeted cryoablative procedures range from direct physical injury to cumulative sublethal cellular stress responses, potentially resulting in molecular-based cell death. The extent of cell injury in response to thermal challenge varies with the time-temperature nature of the treatment. The principles of cryobiology were established with investigations on the treatment of frostbite and with the use of low temperature for pain management, preservation of cells and tissues, and destruction of tumors. This research collectively provides the basis of knowledge needed for the successful utilization of cryotherapy in cardiovascular systems. Application of these principles in research and clinical settings continues to evolve since the onset of the second modern era of cryosurgery in the 1990s, as has our ability to investigate the cellular responses concomitant with the temperature exposure. Selective cryotherapy—the targeting of particular cells or tissues within an organ system—requires an understanding of the mechanisms of injury associated with hypothermic and freezing temperatures. This chapter addresses the interrelated mechanisms of temperature-associated injury and their biological implications.
Mechanical and Molecular Forces
A diverse set of factors associated with low temperature exposure causes cellular and tissue injury, manifested through two primary mechanisms, immediate and delayed injury. Immediate effects including hypothermic damage and freeze rupture are caused by the freezing and thawing of cells. Delayed injury results from vasculature damage and the initiation of necrotic and apoptotic cell death cascades ( Table 2–1 ). Although distinct mechanisms of damage, many direct events exhibit delayed consequences, demonstrating the linkage between the immediate and longer term cell and tissue destruction. These events include, but are not limited to, cellular dehydration, protein denaturation, DNA and RNA damage, alterations in cellular organelle function, uncoupling of biochemical reactions, activation of stress-response cascades, alterations in membrane fluidity, and disruption of ion balance and flow. Each of these events has been reported to be an activator of apoptotic and/or necrotic cell death individually in a strength–duration relation. When activated in combination during freezing, a prolonged molecular-related cell death cascade results. Although lethal intracellular ice is often the focal point of discussion in the application of cryoablation, these molecular-based elements of cellular response are important to consider, particularly in cardiac applications where total cell destruction is not often the ultimate goal, as in cryomapping of arrhythmogenic zones, cryoangioplasty procedures, and using cryotherapy as a palliative pain management tool.
IMMEDIATE IMPACT | DELAYED EFFECTS | |
---|---|---|
Transient | Hypothermic stress | Cumulative hypothermic damage |
Solution effect injury | ||
Permanent | Direct cell injury (ice rupture) | Vascular-mediated injury (necrosis) |
Molecular-mediated death (apoptosis) |
Under normophysiologic conditions, cardiomyocytes exhibit rhythmic action potentials synchronized by the pacemaker cells of the sinoatrial and atrioventricular (AV) nodes, creating the normal cascade of excitatory electrical signals controlling muscular contraction of the heart. As temperature is decreased from 37°C, a series of temperature-dependent mechanical and molecular events occurs. As the tissue temperature approaches mild hypothermia (~32°C), the cell membranes become less fluid, which impacts the transport capacity of the ion pumps and subsequently decreases action potential amplitude, increases its duration, and extends the repolarization period. Cardiac-specific, temperature-dependent, cellular dysfunction has been demonstrated by decreased Ca +2 sensitivity related to the protein kinase C/A phosphorylation of cardiac troponin I (cTn1) in hypothermic cooling and rewarming (ischemia/reperfusion) experiments. As temperature continues to decline, cellular metabolism slows, intracellular pH becomes more acidic, ionic imbalances occur, and energy levels (adenosine triphosphate) decrease. Calcium dysregulation, through ion pump inactivity or failure of the sarcoplasmic reticulum uptake mechanism, may lead to further membrane injury and enzymatic free radical accumulation. The myocardium is particularly sensitive to ischemic injury. The reversibility of these physical alterations acts in an inverse relation to the nadir tissue temperature and temporal duration. The mechanical and molecular effects of hypothermic exposure display both the immediate and delayed modes of cryoinjury. This transient or reversible effect of mild cold on cardiac cells is an important aspect of cryotherapy, distinctive from heat-based thermal therapies. Cryomapping, or the application of mild freezing temperatures to portions of the heart under electrophysiologic monitoring, provides for the identification of arrhythmogenic foci within the myocardium via the transient cessation of cardiac electrical activity within a defined region of the heart. This mapping allows for more precise and selective treatment of cardiac diseases, such as atrial fibrillation.
Ice formation is the cornerstone of tissue injury and occurs both intracellularly and extracellularly. The predominant mode of ice formation is dictated by the freezing rate in conjunction with temperature. The onset of ice formation at the cryoprobe tip provides cryoadhesion to maintain contact between the probe and the tissue throughout the procedure, creating the “heat sink,” the area where heat is extracted from the tissue. As heat is removed by the closed loop circulation of various cryogens, including argon, nitrous oxide, or liquid nitrogen, ice forms in the extracellular spaces and surrounds cells with ice, creating, through freeze concentration of solutes, a hyperosmotic environment. With the withdrawal of water from the cells along these ionic gradients, cellular dehydration occurs, further damaging the membrane and intracellular organelles and macromolecules. Extracellular ice continues to grow in areas experiencing slower rates of cooling and elevated freezing temperatures (tissue distal to the cryoprobe tip). As freezing rates increase and temperatures decrease, the probability of intracellular ice formation increases. The formation of intracellular ice results in the delivery of a lethal condition to the affected area. This region of lethality is typically located within the core of the cryogenic lesion adjacent to the cryoprobe tip. Ablation of arrhythmogenic tissue relies on intracellular freezing. As the distance from the cryoprobe increases, temperatures elevate and the rates of cooling decrease, resulting in a zone of extracellular ice formation where incomplete tissue damage is experienced. Cells and tissue structures farthest from the tip only experience hypothermia, resulting in reversible damage. Although typically considered reversible, the ultimate influence of hypothermic exposure on delayed cell death varies based on exposure duration, severity, and individual cell type sensitivity. During thawing, significant damage also occurs as the ice crystals coalesce into larger crystals, which may also rupture cells ( Table 2–2 ). Thawing of ice produces a transient hypotonic environment in which cells may swell beyond their limits and membranes rupture. Lastly, the eventual return of circulation leads to ischemia-reperfusion injury, which contributes to the activation and manifestation of delayed cell death within the targeted region.
DIRECT CELL DAMAGE | DELAYED VASCULAR DAMAGE | ||
---|---|---|---|
EXTRACELLULAR ICE | INTRACELLULAR ICE | THAWING | MOLECULAR SIGNALING |
|
|
|
|
Freeze/Thaw Cycle
Selection of cryogen defines the nadir temperature that can be reached and the necessary controls for safe delivery to the patient. Recent advances in cryosurgical devices, including a wide range of cryocatheter and cryoprobe designs, provide a number of tools for effective heat transfer. Closed loop systems protect the patient from adverse effects of exposure to the cryogen, relying on the heat transfer properties at the distal end of the probe or catheter to provide the necessary heat sink. Heat is removed in a circumferential manner, creating radial isotherms resulting in a tissue temperature gradient extending outward from the tip. The optimal freeze/thaw cycle consists of a fast cooling rate, ~100°C/min, followed by a slow thawing rate to maximize tissue injury ( Figure 2–1 ). These temperatures and rates are routinely created proximal to the cryoprobe, but rapidly change (elevated freezing temperatures and slower cooling rates) as the distance from the cryoprobe tip increases. The rate of change is a function of both nadir tip temperature and the heat extraction power of the cryogen source ( Table 2–3 ). For example, liquid-based cryogens typically have a greater heat capacity (ability to absorb excess heat) than gas-based cryogens. Furthermore, the extraction of heat by a liquid is more efficient than through a gas interface. This phenomenon can be easily demonstrated by simply placing one hand into cooled air of a refrigerator and submerging the other hand in 4°C water. The hand immersed in the liquid will cool much faster. When developing and applying cryoablation technologies and methods, understanding the heat extraction capacity of the cryogen can be of equal or greater importance than the nadir crotin temperature. Cryoadhesion occurs between the cryoprobe and target tissue at the onset of cryogen flow, maintaining contact, even in the beating heart. Cryoadhesion provides the constant cryoprobe–tissue contact interface necessary for efficient heat transfer and targeted freezing. Cryogenic apparatuses are typically geared toward producing the maximal freezing capacity, based on the cryogen, delivery mode, and probe configurations, to provide adequate thermal contact and tissue ablation. Current cardiac cryoablation devices using pressurized argon or nitrous oxide powered by the Joule–Thomson effect produce a fast cooling rate near the cryotip; however, as a result of the aforementioned thermal limitations, tissues farther from the tip experience much slower cooling rates, approaching 10°C/minute. These warm temperatures and slower cooling rates may result in incomplete cell death.
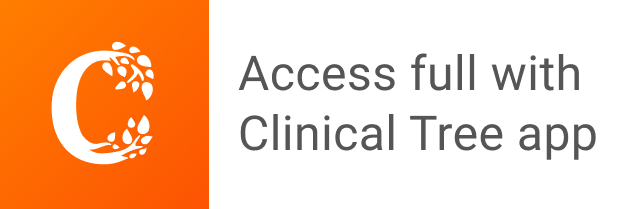