Figure 6–1.
Classification of active cardiac arrhythmias
Abnormal Impulse Formation
Normal Automaticity
Automaticity is the property of cardiac cells to generate spontaneous action potentials. Spontaneous activity is the result of diastolic depolarization caused by a net inward current during phase 4 of the action potential, which progressively brings the membrane potential to threshold (Fig. 6.2). The sino-atrial (SA) node normally displays the highest intrinsic rate. All other pacemakers are referred to as subsidiary or latent pacemakers because they take over the function of initiating excitation of the heart only when the SA node is unable to generate impulses or when these impulses fail to propagate.
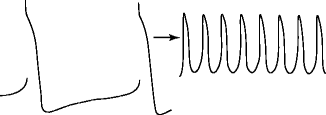
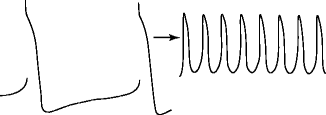
Figure 6–2.
Transition of normal to abnormal automaticity (depolarization-induced low voltage activity) in a Purkinje fiber
The Voltage and Calcium Clocks
Lakatta, Maltsev and their collaborators [1, 2] used the terms sarcolemma voltage clocks and the subsarcolemmal Ca clocks to describe the mechanisms of SA node automaticity. The voltage clock is formed by voltage-sensitive membrane currents, such as the hyperpolarization-activated pacemaker current (I f) [3]. This current is also referred to as a “funny” current because, unlike the majority of voltage-sensitive currents, it is activated by hyperpolarization (from −40/−50 to −100/−110 mV) rather than depolarization. At the end of the action potential, the I f is activated and depolarizes the sarcolemmal membrane [3]. I f is a mixed Na-K inward current modulated by the autonomic nervous system through cAMP. The depolarization activates I Ca,L which provides Ca to activate the cardiac ryanodine receptor (RyR2). The activation of RyR2 initiate sarcoplasmic reticulum (SR) Ca release (Ca induced Ca release), leading to contraction of the heart, a process known as EC coupling. The intracellular Ca (Cai) is then pumped back into SR by the SR Ca-ATPase (SERCA2a) and complete this Ca cycle. In addition to I f, multiple time- and voltage-dependent ionic currents have been identified in cardiac pacemaker cells, which contribute to diastolic depolarization. These currents include (but not limited to) I Ca-L, I Ca-T, I ST, and various types of delayed rectifier K currents [2]. Many of these membrane currents are known to respond to β(beta)-adrenergic stimulation. All these membrane ionic currents contribute to the regulation of SA node automaticity by changing the membrane potential.
Another important ionic current that can depolarize the cell is the sodium-calcium exchanger current (I NCX). In its forward mode, I NCX exchanges three extracellular Na+ with one intracellular Ca2+, resulting in a net intracellular charge gain. This electrogenic current is active during late phase 3 and phase 4 because the Cai decline outlasts the SA node action potential duration. Recent studies showed that I NCX may participate in normal pacemaker activity [4]. The sequence of events includes spontaneous rhythmic SR Ca release, Cai elevation, the activation of I NCX and membrane depolarization. This process is highly regulated by the cAMP and the autonomic nervous system [2]. These studies suggest that sympathetic stimulation accelerates heart rate by phosphorylation of proteins that regulate Cai balance and spontaneous SR Ca cycling. These proteins include phospholamban (PLB, a SR membrane protein regulator of SERCA2a), L-type Ca channels and RyR2. Phosphorylation of these proteins controls the phase and size of subsarcolemmal SR Ca releases. The resulting I NCX contributes to both basal and reserve cardiac pacemaker function.
Subsidiary Pacemakers
In addition to SA node, the atrioventricular (AV) node and Purkinje system are also capable of generating automatic activity. The contribution of I f and I K differs in SA node/AV nodes and Purkinje fiber because of the different potential ranges of these two pacemaker types (i.e., −70 to −35 mV and −90 to −65 mV, respectively). The contribution of other voltage-dependent currents can also differ among the different cardiac cell types. Whether or not Ca clock plays a role in pacemaking of AV node and Purkinje cells remain unclear.
Cells in the SA node possess the fastest intrinsic rates. Thus the SA node is the primary pacemaker in the normal heart. When impulse generation or conduction within or out of the SA node is impaired, latent or subsidiary pacemakers within the atria or ventricles take control of pacing the heart. The intrinsically slower rates of these latent pacemakers generally result in bradycardia. Both atrial and AV junctional subsidiary pacemakers are under autonomic control, with the sympathetic system increasing and parasympathetic system slowing the pacing rate. Although acetylcholine produces little in the way of a direct effect, it can significantly reduce Purkinje automaticity by means of the inhibition of the sympathetic influence, a phenomenon termed accentuated antagonism [5]. Simultaneous recording of cardiac sympathetic and parasympathetic activity in ambulatory dogs confirmed that sympathetic activation followed by vagal activation may be associated with significant bradycardia [6, 7].
Automaticity as a Mechanism of Cardiac Arrhythmias
Abnormal automaticity includes both reduced automaticity, which causes bradycardia, and increased automaticity, which causes tachycardia. Arrhythmias caused by abnormal automaticity can result from diverse mechanisms (see Figs. 6.1 and 6.2). Alterations in sinus rate can be accompanied by shifts of the origin of the dominant pacemaker within the sinus node or to subsidiary pacemaker sites elsewhere in the atria. Impulse conduction out of the SA mode can be impaired or blocked as a result of disease or increased vagal activity leading to development of bradycardia. AV junctional rhythms occur when AV junctional pacemakers located either in the AV node or in the His bundle accelerate to exceed the rate of SA node, or when the SA nodal activation rate was too slow to suppress the AV junctional pacemaker.
Hereditary Bradycardia
Bradycardia can occur in structurally normal hearts due to genetic mutations that result in abnormalities of either membrane clock or Ca clock mechanisms of automaticity. One example is the mutation of hyperpolarization-activated nucleotide-gated channel (HCN4), which is part of the channels that carry I f. Mutations of the HCN4 may cause familial bradycardia as well [8, 9].
Secondary SA Node Dysfunction
Common diseases, such as heart failure and atrial fibrillation, may be associated with significant SA node dysfunction. Malfunction of both membrane voltage clocks and Ca clocks might be present in both of these common diseases. Zicha et al. [10] reported downregulation of HCN4 expression contributes to heart failure-induced sinus node dysfunction. An A450V missense loss of function mutation in HCN4 has recently been shown to underlie familial sinus bradycardia in several unrelated proband of Moroccan Jewish descent [9, 11–13].
Enhanced Automaticity
Atrial and ventricular myocardial cells do not display spontaneous diastolic depolarization or automaticity under normal conditions, but can develop these characteristics when depolarized, resulting in the development of repetitive impulse initiation, a phenomenon termed depolarization-induced automaticity (see Fig. 6.2) [14]. The membrane potential at which abnormal automaticity develops ranges between −70 and −30 mV. The rate of abnormal automaticity is substantially higher than that of normal automaticity and is a sensitive function of resting membrane potential (i.e., the more depolarized resting potential the faster the rate). Similar to normal automaticity, abnormal automaticity is enhanced by β(beta)-adrenergic agonists and by reduction of external potassium.
Depolarization of membrane potential associated with disease states is most commonly a result of either: (1) an increase in extracellular potassium, which reduces the reversal potential for I K1, the outward current that largely determines the resting membrane or maximum diastolic potential; (2) a reduced number of IK1 channels; (3) a reduced ability of the IK1 channel to conduct potassium ions; or (4) electrotonic influence of neighboring cells in the depolarized zone. Because the conductance of I K1 channels is a sensitive to extracellular potassium concentration, hypokalemia can lead to major reduction in I K1, leading to depolarization and the development of enhanced or abnormal automaticity, particularly in Purkinje pacemakers. A reduction in I K1 can also occur secondary to a mutation in KCNJ2, the gene that encodes for this channel, leading to increased automaticity and extrasystolic activity presumably arising from the Purkinje system [15, 16]. Loss of function KCNJ2 mutation gives rise to Andersen-Tawil syndrome, which is characterized among other things by a marked increase in extrasystolic activity [17–19].
Overdrive Suppression of Automaticity
The automaticity of most pacemakers within the heart is inhibited when they are overdrive paced [20]. This inhibition is called overdrive suppression. Under normal condition all subsidiary pacemakers are overdrive-suppressed by SA nodal activity. A possible mechanism of overdrive suppression is intracellular accumulation of Na leading to enhanced activity of the sodium pump (sodium-potassium adenosine triphosphatase [Na+-K+ ATPase]), which generates a hyperpolarizing electrogenic current that opposes phase 4 depolarization [21]. The faster the overdrive rate or the longer the duration of overdrive, the greater the enhancement of sodium pump activity, so that the period of quiescence after cessation of overdrive is directly related to the rate and duration of overdrive.
Parasystole and Modulated Parasystole
Latent pacemakers throughout the heart are generally reset by the propagating wavefront initiated by the dominant pacemaker. An exception to this rule occurs when the pacemaking tissue is protected from the impulse of sinus nodal origin. A region of entrance block arises when cells exhibiting automaticity are surrounded by ischemic, infarcted, or otherwise compromised cardiac tissues that prevent the propagating wave from invading the focus, but which permit the spontaneous beat generated within the automatic focus to exit and activate the rest of the myocardium. A pacemaker region exhibiting entrance block, and exit conduction is referred to as a parasystolic focus. The ectopic activity generated by a parasystolic focus is characterized by premature ventricular complexes with variable coupling intervals, fusion beats and inter-ectopic intervals that are multiples of a common denominator. This rhythm is relatively rare and is usually considered benign, although a premature ventricular activation of parasystolic origin can induce malignant ventricular rhythms in the ischemic myocardium or in the presence of a suitable myocardial substrate.
Modulated parasystole, a variant of classical parasystole, was described by Moe and co-workers [22, 23]. This variant of the arrhythmia results from incomplete entrance block of the parasystolic focus. Electrotonic influences arriving early in the pacemaker cycle delayed and those arriving late in the cycle accelerated the firing of the parasystolic pacemaker, so that ventricular activity could entrain the partially protected pacemaker. As a consequence, at select heart rate, extrasystolic activity generated by the entrained parasystolic pacemaker can mimic reentry, generating extrasystolic activity with fixed coupling [22–26].
Afterdepolarization and Triggered Activity
Oscillatory depolarizations that attend or follow the cardiac action potential and depend on preceding transmembrane activity for their manifestation are referred to as afterdepolarizations (Figs. 6.3, 6.4, and 6.5). Two subclasses traditionally recognized: (1) early, and (2) delayed. Early afterdepolarization (EADs) interrupt or retard repolarization during phase 2 and/or phase 3 of the cardiac action potential, whereas delayed afterdepolarization (DADs) occur after full repolarization. When EAD or DAD amplitude suffices to bring the membrane to its threshold potential, a spontaneous action potential referred to as a triggered response is the result (see Figs. 6.3, 6.4, and 6.5). These triggered events giver rise to extrasystoles, which can precipitate rapid cardiac arrhythmias.
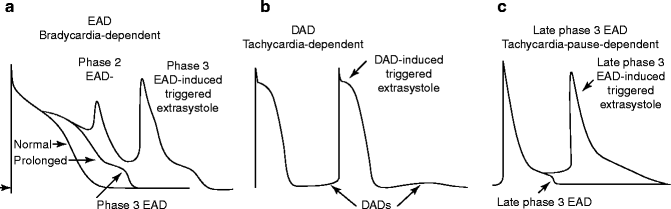
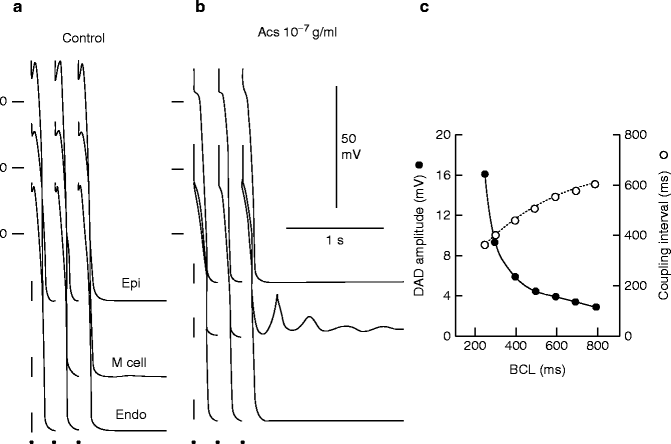
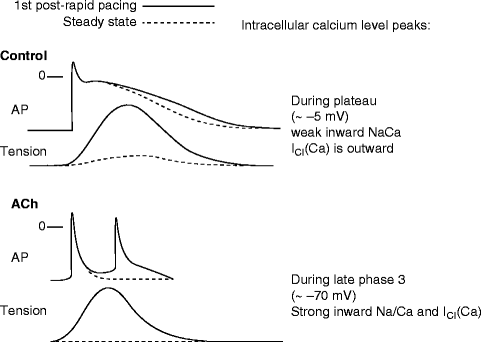
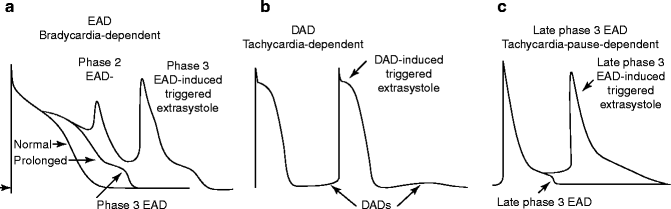
Figure 6–3.
Early (EAD) and delayed (DAD) afterdepolarizations and EAD- and DAD-induced triggered action potentials (AP). (a) Phase 2 EAD and phase 3 EAD-induced APs in canine isolated Purkinje fiber preparation treated with d-sotalol (IKr block). The conditional phase of EAD is defined as the time interval spanning from the moment when membrane potential starts to deviate from normal coarse to the moment that immediately precedes the EAD upstroke or down-stroke. (b) DAD- and DAD-induced triggered activity in canine ventricular preparation induced by rapid pacing in the presence of isoproterenol (β-adrenergic agonist, augmenting intracellular calcium activity). (c) Late phase 3 EAD-induced triggered beat in canine right atrium in the condition of abbreviated repolarization (in the presence of acetylcholine, a parasympathetic agonist). Shown are the first sinus beat and following late phase 3 EAD-inducing triggered beat after a period of rapid activation (Panels a and b reproduced from [27] with permission from John Wiley and Sons, and panel c from [28] with permission from Wolters Kluwer Health)
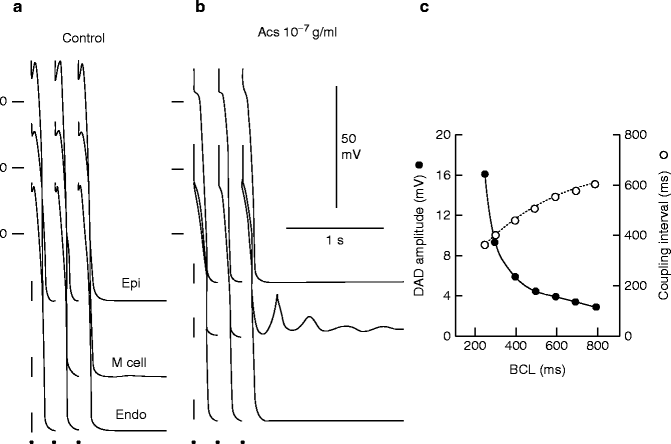
Figure 6–4.
Digitalis-induced delayed afterdepolarizations in M cells but not epicardium or endocardium. Effects of acetylstrophanthidin (AcS) on transmembrane activity of an epicardial (Epi), endocardial (Endo) and M cell preparation. [K+]o = 4 mM. (a) Control. (b) Recorded after 90 min of exposure to 10−7 g/ml AcS. Each panel shows the last three beats of a train of ten basic beats elicited at a basic cycle length (BCL) of 250 ms. Each train is followed by a 3 s pause. AcS induced prominent delayed afterdepolarizations (DADs) in the M cell preparation but not in epicardium or endocardium. (c) Rate-dependence of coupling interval and amplitude of the AcS-induced DADs. Measured is the first DAD recorded from the M cell (From [29] with permission from John Wiley and Sons)
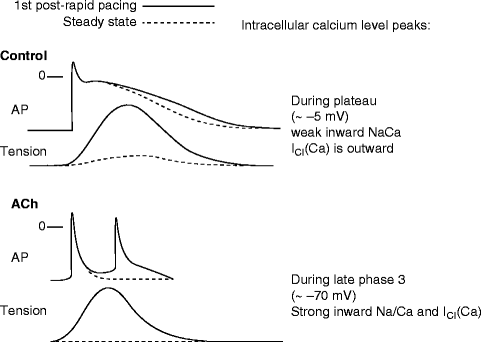
Figure 6–5.
Proposed mechanism for the development of late phase 3 EADs. Shown are superimposed action potential (AP) and phasic tension recordings obtained under steady state conditions and during the first regular post-rapid pacing beat in control and in the presence of acetylcholine. See text for further discussion (Reproduced from [28] with permission from Wolters Kluwer Health)
Early Afterdepolarizations and Triggered Activity
EADs are observed in isolated cardiac tissues exposed to injury, altered electrolytes, hypoxia, acidosis, catecholamines, and pharmacologic agents, including antiarrhythmic drugs. Ventricular hypertrophy and heart failure also predispose to the development of EADs [30]. EAD characteristics vary as a function of animal species, tissue or cell type, and the method by which the EAD is elicited. Although specific mechanisms of EAD induction can differ, a critical prolongation of repolarization accompanies most, but not all, EADs. Drugs that inhibit potassium currents or which augment inward currents predispose to the development of EADs [31]. Phase 2 and phase 3 EADs sometimes appear in the same preparation.
EAD-induced triggered activity is sensitive to stimulation rate. Antiarrhythmic drugs with class III action generally induce EAD activity at slow stimulation rates [14]. In contrast, β(beta)-adrenergic agonist–induced EADs are fast rate-dependent [32]. In the presence of rapidly activating delayed rectifier current (rapid outward potassium current [I Kr]) blockers, β(beta)-adrenergic agonists, and/or acceleration from an initially slow rate transiently facilitate the induction of EAD activity in ventricular M cells, but not in epicardium or endocardium and rarely in Purkinje fibers [33].
Cellular Origin of Early Afterdepolarizations
EADs develop more commonly in midmyocardial M cells and Purkinje fibers than in epicardial or endocardial cells when exposed to APD-prolonging agents. This is due to the presence of a weaker I Ks and stronger late INa in M cells [34, 35]. Block of I Ks with chromanol 293B permits the induction of EADs in canine epicardial and endocardial tissues in response to I Kr blockers such as E-4031 or sotalol [36]. The predisposition of cardiac cells to the development of EADs depends principally on the reduced availability of I Kr and I Ks as occurs in many forms of cardiomyopathy. Under these conditions, EADs can appear in any part of the ventricular myocardium [37].
Ionic Mechanisms Responsible for the EAD
EADs develop when the balance of current active during phase 2 or 3 of the action potential shifts in the inward direction. If the change in current–voltage relation results in a region of net inward current during the plateau range of membrane potentials, it leads to a depolarization or EAD. Most pharmacological interventions or pathophysiological conditions associated with EADs can be categorized as acting predominantly through one of four different mechanisms: (1) A reduction of repolarizing potassium currents (I Kr, class IA and III antiarrhythmic agents; I Ks, chromanol 293B or I K1); (2) an increase in the availability of calcium current (Bay K 8644, catecholamines); (3) an increase in the sodium-calcium exchange current (I NCX) caused by augmentation of Cai activity or upregulation of the I NCX; and (4) an increase in late sodium current (late I Na) (aconitine, anthopleurin-A, and ATX-II). Combinations of these interventions (i.e., calcium loading and IKr reduction) or pathophysiological states can act synergistically to facilitate the development of EADs.
Delayed Afterdepolarization-Induced Triggered Activity
DADs and DAD-induced triggered activity are observed under conditions that increase intracellular calcium, [Ca2+]i, such as after exposure to toxic levels of cardiac glycosides (digitalis) [38–40] or catecholamines [32, 41, 42]. This activity is also manifest in hypertrophied and failing hearts [43, 44] as well as in Purkinje fibers surviving myocardial infarction [45]. In contrast to EADs, DADs are always induced at relatively rapid rates. Recent studies have shown that ouabain-induced activation of calcium calmodulim-dependent protein kinase II (CaMKII) gives rise to an augmentation of late INa, thus contributing to the development of calcium overload and digitalis toxicity [46]. Ranolazine, a late INa blocker, prevented the ouabain-induced Ca overload and toxic manifestations. Yao and co-workers further showed that Nav1.5-dependent increase in sodium influx leads to activation of CaMKII, which in turn phosphorylates Nav1.5, further promoting sodium influx and that inhibition of either CaMKII or Nav1.5 can ameliorate cardiac dysfunction associated with excessive sodium influx [47].
Role of Delayed Afterdepolarization-Induced Triggered Activity in the Development of Cardiac Arrhythmias
An example of DAD-induced arrhythmia is the catecholaminergic polymorphic ventricular tachycardia (CPVT), which may be caused by the mutation of either the type 2 ryanodine receptor (RyR2) or the calsequestrin (CSQ2) [48]. The principal mechanism underlying these arrhythmias is the “leaky” ryanodine receptor, which is aggravated during catecholamine stimulation. A typical clinical phenotype of CPVT is bidirectional ventricular tachycardia, which is also seen in digitalis toxicity. Wehrens et al. [49] demonstrated that heterozygous mutation of FKBP12.6 leads to leaky RyR2 and exercise-induced VT and VF, simulating the human CPVT phenotype. RyR2 stabilization with a derivative of 1,4-benzothiazepine (JTV519) increased the affinity of calstabin2 for RyR2, which stabilized the closed state of RyR2 and prevented the Ca leak that triggers arrhythmias. Other studies indicate that delayed afterdepolarization-induced extrasystoles serve to trigger catecholamine-induced VT/VF, but that the epicardial origin of these ectopic beats increases transmural dispersion of repolarization, thus providing the substrate for the development of reentrant tachyarrhythmias, which underlie the rapid polymorphic VT/VF [50]. Heart failure is associated with structural and electrophysiological remodeling, leading to tissue heterogeneity that enhances arrhythmogenesis and the propensity of sudden cardiac death [51].
Late Phase 3 Early Afterdepolarizations and Their Role in the Initiation of Fibrillation
In 2003, Burashnikov and Antzelevitch described a novel mechanism giving rise to triggered activity, termed “late phase 3 EAD”, which combines properties of both EAD and DAD, but has its own unique character (see Figs. 6.3 and 6.5) [27, 28]. Late phase 3 EAD-induced triggered extrasystoles represent a new concept of arrhythmogenesis in which abbreviated repolarization permits “normal SR calcium release” to induce an EAD-mediated closely coupled triggered response, particularly under conditions permitting intracellular calcium loading [27, 28]. These EADs are distinguished by the fact that they interrupt the final phase of repolarization of the action potential (late phase 3). In contrast to previously described DAD or Cai-depended EAD, it is normal, not spontaneous SR calcium release that is responsible for the generation of the EAD. Two principal conditions are required for the appearance of late phase 3 EAD: an APD abbreviation and a strong SR calcium release [28]. Such conditions may occur when both parasympathetic and sympathetic influences are combined. Simultaneous sympathovagal activation is also known to be the primary trigger of paroxysmal atrial tachycardia and AF episodes in dogs with intermittent rapid pacing [6].
Late phase 3 EAD-induced extrasystoles have been shown to initiate AF in canine atria, particularly following spontaneous termination of the arrhythmia (IRAF, immediate reinduction of AF) [28]. The appearance of late phase 3 EAD immediately following termination of AF or rapid pacing has been reported by in the canine atria in vivo[52] and pulmonary veins in vitro [53]. The pattern of initiation of some forms of paroxysmal AF in humans (i.e., tachycardia- or pause- dependent AF initiation) is consistent with behavior of late phase 3 EAD [54]. In addition to the atrial arrhythmias, late phase 3 EAD may also be responsible for the development recurrent VF in failing hearts [55].
Reentrant Arrhythmias
Reentry is fundamentally different from automaticity or triggered activity in the mechanism by which it initiates and sustains cardiac arrhythmias. Circus movement reentry occurs when an activation wavefront propagates around an anatomical or functional obstacle or core, and reexcites the site of origin (Fig. 6.6). In this type of reentry, all cells take turns in recovering from excitation so that they are ready to be excited again when the next wavefront arrives. In contrast, reflection and phase 2 reentry occur in a setting in which large differences of recovery from refractoriness exists between one site and another. The site with delayed recovery serves as a virtual electrode that excites its already recovered neighbor, resulting in a reentrant re-excitation. In addition, reentry can also be classified as anatomical and functional, although there is a grey zone in which both functional and anatomical factors are important in determining the characteristics of reentrant excitation.
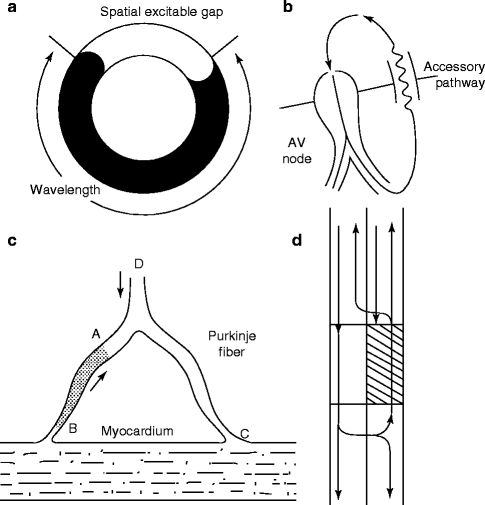
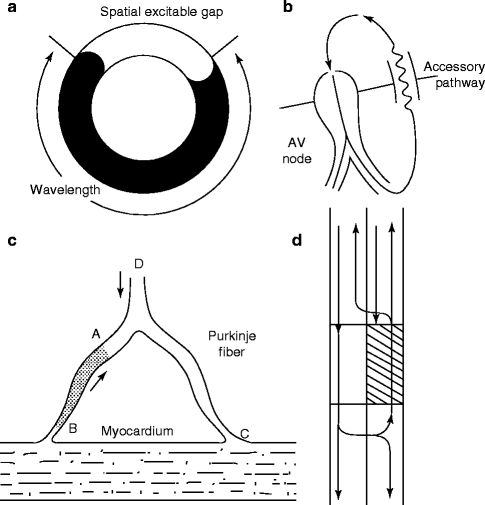
Figure 6–6.
Ring models of reentry. (a) Schematic of a ring model of reentry. (b) Mechanism of reentry in the Wolf-Parkinson-White syndrome involving the AV node and an atrioventricular accessory pathway (AP). (c) A mechanism for reentry in a Purkinje-muscle loop proposed by Schmitt and Erlanger. The diagram shows a Purkinje bundle (D) that divides into two branches, both connected distally to ventricular muscle. Circus movement was considered possible if the stippled segment, A → B, showed unidirectional block. An impulse advancing from D would be blocked at A, but would reach and stimulate the ventricular muscle at C by way of the other terminal branch. The wavefront would then reenter the Purkinje system at B traversing the depressed region slowly so as to arrive at A following expiration of refractoriness. (d) Schematic representation of circus movement reentry in a linear bundle of tissue as proposed by Schmitt and Erlanger. The upper pathway contains a depressed zone (shaded) serves as a site of unidirectional block and slow conduction. Anterograde conduction of the impulse is blocked in the upper pathway but succeeds along the lower pathway. Once beyond the zone of depression, the impulse crosses over through lateral connections and reenters through the upper pathway (Panels c and d are from Schmitt and Erlanger [56], reprinted with permission from Springer Science + Business Media)
Circus Movement Reentry Around an Anatomical Obstacle
The ring model is the prototypical example of reentry around an anatomical obstacle (see Fig. 6.6). It first emerged as a concept shortly after the turn of the last century when Mayer reported the results of experiments involving the subumbrella tissue of a jellyfish (Sychomedusa cassiopeia) [57]. The muscular disk did not contract until ringlike cuts were made and pressure and a stimulus applied. This caused the disc to “spring into rapid rhythmical pulsation so regular and sustained as to recall the movement of clockwork.” Mayer demonstrated similar circus movement excitation in rings cut from the ventricles of turtle hearts, but he did not consider this to be a plausible mechanism for the development of cardiac arrhythmias. His experiments proved valuable in identifying two fundamental conditions necessary for the initiation and maintenance of circus movement excitation: (1) unidirectional block—the impulse initiating the circulating wave must travel in one direction only; and (2) for the circus movement to continue, the circuit must be long enough to allow each site in the circuit to recover before the return of the circulating wave. Mines [58] was the first to develop the concept of circus movement reentry as a mechanism responsible for cardiac arrhythmias. He confirmed Mayer’s observations and suggested that the recirculating wave could be responsible for clinical cases of tachycardia [59]. The following three criteria developed by Mines for identification of circus movement reentry remains in use today:
1.
An area of unidirectional block must exist.
2.
The excitatory wave progresses along a distinct pathway, returning to its point of origin and then following the same path again.
3.
Interruption of the reentrant circuit at any point along its path should terminate the circus movement.
It was recognized that successful reentry could occur only when the impulse was sufficiently delayed in an alternate pathway to allow for expiration of the refractory period in the tissue proximal to the site of unidirectional block. Both conduction velocity and refractoriness determine the success or failure of reentry, and the general rule is that the length of the circuit (pathlength) must exceed or equal that of the wavelength, the wavelength being defined as the product of the conduction velocity and the refractory period or that part of the pathlength occupied by the impulse and refractory to reexcitation. The theoretical minimum path length required for development of reentry was therefore dependent on both the conduction velocity and the refractory period. Reduction of conduction velocity or APD can both significantly reduce the theoretical limit of the pathlength required for the development or maintenance of reentry.
Circus Movement Reentry Without an Anatomical Obstacle
In 1924, Garrey suggested that reentry could be initiated without the involvement of anatomic obstacles and that “natural rings are not essential for the maintenance of circus contractions” [60]. Nearly 50 years later, Allessie and coworkers [61] provided direct evidence in support of this hypothesis in experiments in which they induced a tachycardia in isolated preparations of rabbit left atria by applying properly timed premature extra-stimuli (Fig. 6.7). Using multiple intracellular electrodes, they showed that although the basic beats elicited by stimuli applied near the center of the tissue spread normally throughout the preparation, premature impulses propagate only in the direction of shorter refractory periods. An arc of block thus develops around which the impulse is able to circulate and reexcite its site of origin. Recordings near the center of the circus movement showed only subthreshold responses. The authors proposed the term “leading circle” to explain their observation [62]. They argued that the functionally refractory region that develops at the vortex of the circulating wavefront prevents the centripetal waves from short circuiting the circus movement and thus serves to maintain the reentry. The authors also propose that the refractory core was maintained by centripetal wavelets that collide with each other. Because the head of the circulating wavefront usually travels on relatively refractory tissue, a fully excitable gap of tissue may not be present; unlike other forms of reentry the leading circle model may not be readily influenced by extraneous impulses initiated in areas outside the reentrant circuit and thus may not be easily entrained. Although the leading circle reentry for a while was widely accepted as a mechanism of functional reentry, there is significant conceptual limitation to this model of reentry. For example, the centripetal wavelet was difficult to demonstrate either by experimental studies with high resolution mapping or with computer simulation studies.
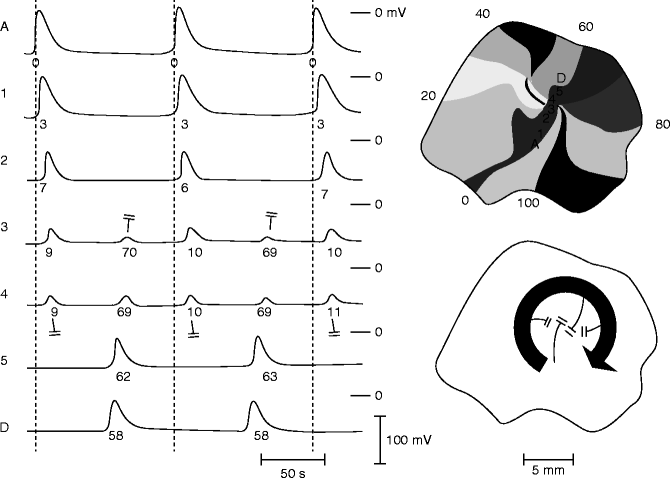
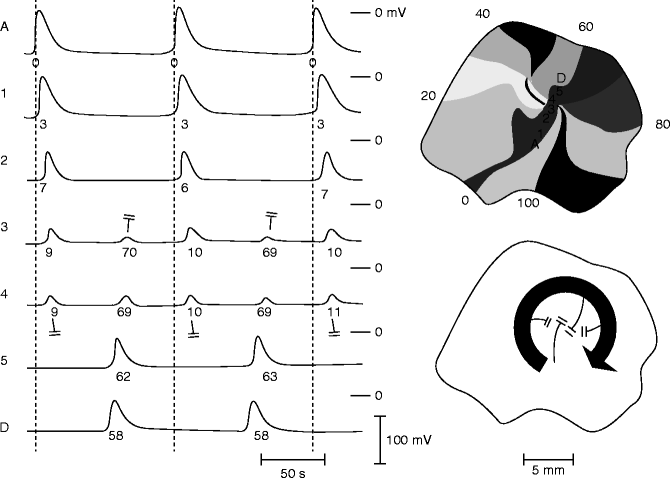
Figure 6–7.
Leading circle model of reentry. Activation maps during steady-state tachycardia induced by a premature stimulus in an isolated rabbit atrium (upper right). On the left are transmembrane potentials recorded from seven fibers located on a straight line through the center of the circus movement. Note that the central area is activated by centripetal wavelets and that the fibers in the central area show double responses of subnormal amplitude. Both responses are unable to propagate beyond the center, thus preventing the impulse from short-cutting the circuit. Lower right: the activation pattern is schematically represented, showing the leading circuit and the converging centripetal wavelets. Block is indicated by double bars (From Allessie et al. [62] with permission from Wolters Kluwer Health)
First introduced by Rosenblueth and Weiner in 1946 [63], the concept of spiral waves (rotors) has attracted a great deal of interest over the past decade. Originally used to describe reentry around an anatomic obstacle, the term spiral wave reentry was later adopted to describe circulating waves in the absence of an anatomic obstacle [64, 65]. Because spiral waves of excitation is a well described phenomenon in many excitable media [66], the application of the spiral waves of excitation to cardiac tissues is met with great enthusiasm. Spiral wave theory has advanced our understanding of the mechanisms responsible for the functional form of reentry. Although leading circle and spiral wave reentry are considered by some to be similar, a number of distinctions have been suggested. The curvature of the spiral wave is the key to the formation of the core [67]. The curvature of the wave forms a region of high impedance mismatch (source-sink mismatch), where the current provided by the reentering wavefront (source) is insufficient to charge the capacity and thus excite larger volume of tissue ahead (sink). A prominent curvature of the spiral wave is generally encountered following a wave break, where the wavefront meets the wavetail and a large curvature (and short action potential) is present. Due to a very small source in part related to a short action potential (wavefront and wavetail meets), the broken end of the wave moves most slowly. Figure 6.8 shows the formation of the spiral wave by wave front interaction with the refractory tail of a previous activation [70]. This three dimensional computer simulation study reproduced the wavebreak observed in the optical mapping studies of VF in swine ventricle. The wavebreak occurs when the wavefront encountered refractory tail of a previous activation, inducing two spiral waves (Panel A). A three dimensional view of the scroll wave is shown in Panel B. Panel C is a blow up of the wavebreak. Note that the newly formed wavebreak has a very high curvature. As curvature decreases along the more distal parts of the spiral, propagation speed increases. The high curvature prevents the wave from propagating in the direction of wavebreak. The wavefront then circles around the wavebreak site to form circus movement. In three dimensions, there are two new scroll waves formed by these interactions. Another difference between the leading circle and spiral wave is the state of the core; in former the core is refractory because of repetitive centripetal wavelet that invades the core. In latter the core remains unexcited because the source-sink mismatch prevented the propagation of the wavefront into the core.
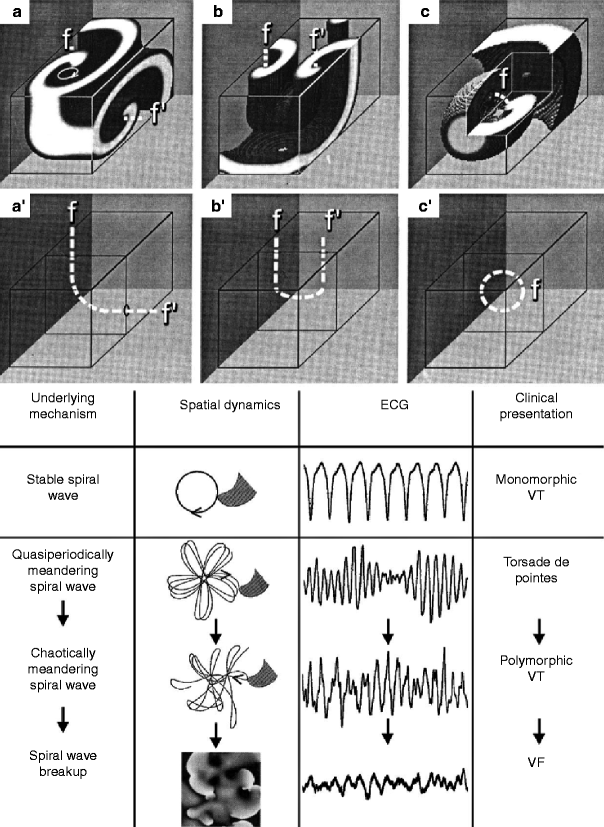
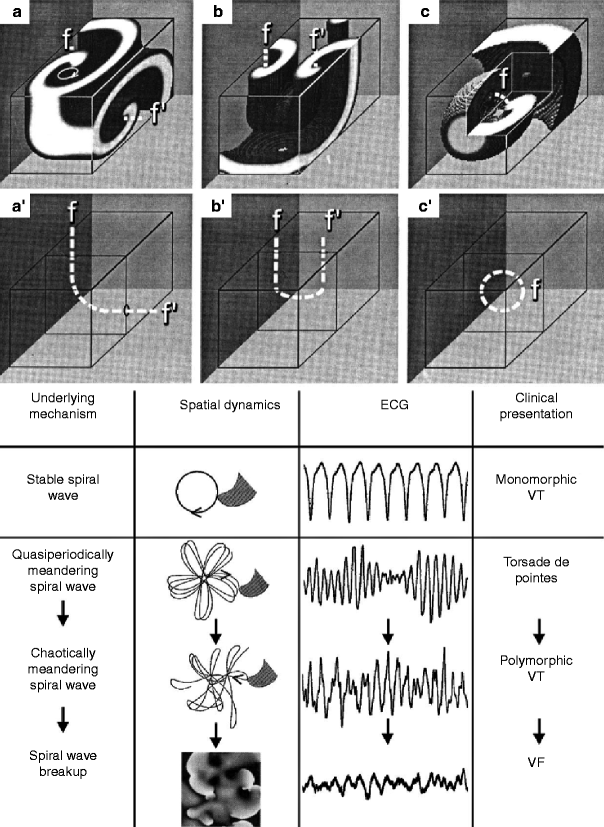
Figure 6–8.
Schematic representation of basic scroll-type reentry in 3-D and spiral wave phenotypes with their possible clinical manifestations. Upper panel: Basic configurations of vortex-like reentry in three dimensions. (a, a’) L-shaped scroll wave and filament, respectively. The scroll rotates in a clockwise direction (on the top) about the L-shaped filament (f, f’) shown in (a’). (b, b’) U-shaped scroll wave and filament, respectively. (c, c’) O-shaped wave and filament, respectively (From Pertsov and Jalife [68] with permission). Bottom panel: Four types of spiral wave phenotypes and associated clinical manifestations. A stable spiral wave mechanism gives rise to monomorphic ventricular tachycardia (VT) on the ECG. A quasi-periodic meandering spiral wave is responsible for Torsade de Pointes, whereas a chaotically meandering spiral wave is revealed as polymorphic VT. A ventricular fibrillation (VF) pattern is caused by spiral wave breakup. Second column, spiral wave are shown in gray; the path of their tip are shown as solid lines (From Garfinkel and Qu [69], with permission)
The term spiral wave is usually used to describe reentrant activity in two dimensions. The center of the spiral wave is called the core and the distribution of the core in three dimensions is referred to as the filament. The three-dimensional form of the spiral wave forms a scroll wave (see Fig. 6.8b). In its simplest form, the scroll wave has a straight filament spanning the ventricular wall (i.e., from epicardium to endocardium). Theoretical studies have described three major scroll wave configurations with curved filaments (L-, U-, and O-shaped), although numerous variations of these three-dimensional filaments in space and time are assumed to exist during cardiac arrhythmias.
Spiral wave activity has been used to explain the electrocardiographic patterns observed during monomorphic and polymorphic cardiac arrhythmias as well as during fibrillation. Monomorphic VT results when the spiral wave is anchored and not able to drift within the ventricular myocardium. In contrast, a meandering or drifting spiral wave causes polymorphic VT and VF like activity [71]. VF seems to be the most complex representation of rotating spiral waves in the heart. VF is often preceded by VT. One of the theories suggests that VF develops when a single spiral wave responsible for VT breaks up, leading to the development of multiple spirals that are continuously extinguished and re-created [72].
Figure-Eight Reentry
In the late 1980s, El-Sherif and coworkers delineated a figure-eight reentry in the surviving epicardial layer overlying an area of infarction produced by occlusion of the left anterior descending artery in canine hearts [73]. The same patterns of activation can also be induced by creating artificial anatomical obstacles in the ventricles [74], or during functional reentry induced by a single premature ventricular stimulation [75]. In the figure-eight model, the reentrant beat produces a wavefront that circulates in both directions around a line of conduction block rejoining on the distal side of the block. The wavefront then breaks through the arc of block to reexcite the tissue proximal to the block. The reentrant activation continues as two circulating wavefronts that travel in clockwise and counterclockwise directions around the two arcs in a pretzel-like configuration. Figure-eight reentry can be anatomical, functional, or spiral wave reentry. Thus, figure-eight reentry is a two-dimensional representation of all possible spatiotemporal representations of these reentrant mechanisms.
Cardiac Fibrillation: Multiple Wavelets or Single Source?
There are two major theories to explain AF/VF generation. The first, originally suggested by Gordon Moe and colleagues, proposes that cardiac fibrillation is maintained by multiple unstable reentrant wavelets [76]. The multiple reentrant wavelet hypothesis of cardiac fibrillation was the dominant theory from 1960 to 1990. Starting in the 1980’s, studies employing activation mapping techniques provided evidence for the presence of multiple reentrant wavelets during atrial and ventricular fibrillation [72, 77–80]. Starting in the 1990s, with a growing number of groups conducting mapping studies as well as with an improvement of mapping technologies, it became evident that multiple reentrant wavelets could not be readily observed during fibrillation. In fact, results of most mapping studies revealed that during fibrillation, a full reentrant circuit is either not recorded at all, observed rarely as a single short-lived rotor, or manifest as a single stable or meandering rapidly revolving micro-reentrant circuit, leading to disorganized activity due to fibrillatory conduction in the rest of the atria or ventricles [71, 81–90]. These data promoted the revival of another theory for the maintenance of VF/AF known as the “single source hypothesis”, which was first suggested 50 years earlier by Scherf et al. [91] and Prinzmetal et al. [92]. This theory proposes that AF/VF can be maintained by a single high frequency source, giving rise to impulse propagation with variable conduction block in the remainder of the ventricle (i.e., fibrillatory conduction), which accounts for the AF/VF pattern in the ECG. Jalife and co-workers were among the strongest advocates for the single source hypothesis, with a “mother rotor” being the driving force for AF/VF [87, 88, 93].
Most of the fibrillation activation mapping data have been obtained from epicardial and/or endocardial surfaces, where a full reentrant circuit is not readily observed [71, 81–85, 90, 94, 95]. A common interpretation of these results is that reentrant circuit(s) or part(s) of the circuit(s) travels intramurally, and, thus, may not be readily observed on the cardiac surfaces. Indeed, some of the studies that used both epicardial and endocardial mapping have revealed that a focal pattern of activation on the surface can be due to a reentrant circuit involving epicardial, intramural, and endocardial pathways [96, 97]. Some studies using intramural plunge electrode mapping techniques presented evidence for intramural scroll waves during VF [78, 90]. Factors that reduce the chance of detecting driver(s) of AF/VF include limited mapping resolution and/or cardiac area available for mapping. Taking into account these limitations and given that AF/VF generation may involve a complex 3-D spatiotemporal electrical activation (even in the case of relatively thin atrial structures [96, 98]), it is often difficult to decisively prove or disprove the presence of a single or multiple sources. Moreover, while a reentrant mechanisms are believed to be primary, a rapid focal source may also maintain some forms of atrial and ventricular fibrillation [83, 99–103]. While the weight of available evidence appears to point to a single driving source as the primary mechanism for the maintenance of AF/VF [90, 93], it is well recognized that mechanisms of cardiac fibrillation are unlikely to be the same in all cases, and that fibrillations may be maintained by diverse mechanisms [93, 97, 100, 104].
Reflection
Reentry can occur without circus movement. Reflection and phase 2 reentry are two examples of non-circus movement reentry. The concept of reflection was first suggested by studies of the propagation characteristics of slow action potential responses in K+-depolarized Purkinje fibers [105]. In strands of Purkinje fiber, Wit and coworkers demonstrated a phenomenon similar to that observed by Schmitt and Erlanger in which slow anterograde conduction of the impulse was at times followed by a retrograde wavefront that produced a “return extrasystole” [105]. They proposed that the nonstimulated impulse was caused by circuitous reentry at the level of the syncytial interconnections, made possible by longitudinal dissociation of the bundle, as the most likely explanation for the phenomenon but also suggested the possibility of reflection. Direct evidence in support of reflection as a mechanism of arrhythmogenesis was provided by Antzelevitch and coworkers in the early 1980s [106, 107]. A number of models of reflection have been developed. The first of these involves use of ion-free isotonic sucrose solution to create a narrow (1.5–2 mm) central inexcitable zone (gap) in unbranched Purkinje fibers mounted in a three-chamber tissue bath (Fig. 6.9) [108]. In the sucrose-gap model, stimulation of the proximal (P) segment elicits an action potential that propagates to the proximal border of the sucrose gap. Active propagation across the sucrose gap is not possible because of the ion-depleted extracellular milieu, but local circuit current continues to flow through the intercellular low resistance pathways (an Ag/AgCl extracellular shunt pathway is provided). This local circuit or electrotonic current, very much reduced on emerging from the gap, gradually discharges the capacity of the distal (D) tissue thus giving rise to a depolarization that manifests as a either a sub-threshold response (last distal response) or a foot-potential that brings the distal excitable tissue to its threshold potential. Active impulse propagation stops and then resumes after a delay that can be as long as several 100 ms. When anterograde (P–D) transmission time is sufficiently delayed to permit recovery of refractoriness at the proximal end, electrotonic transmission of the impulse in the retrograde direction is able to reexcite the proximal tissue, thus generating a closely coupled reflected reentry. Reflection therefore results from the to-and-fro electrotonically-mediated transmission of the impulse across the same in-excitable segment; neither longitudinal dissociation nor circus movement need be invoked to explain the phenomenon.
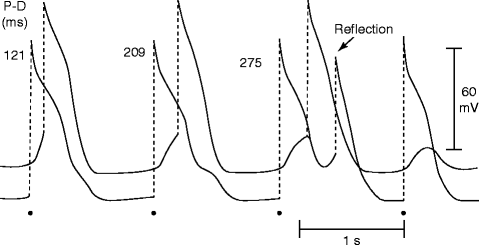
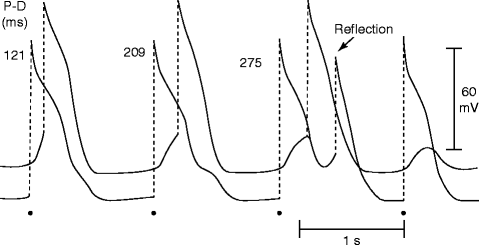
Figure 6–9.
Delayed transmission and reflection across an inexcitable gap created by superfusion of the central segment of a Purkinje fiber with an ion-free isotonic sucrose solution. The two traces were recorded from proximal (P) and distal (D) active segments. P–D conduction time (indicated in the upper portion of the figure, in ms) increased progressively with a 4:3 Wenckebach periodicity. The third stimulated proximal response was followed by a reflection (From Antzelevitch et al [108], with permission from Elsevier Limited)
A second model of reflection involved the creation of an inexcitable zone permitting delayed conduction by superfusion of a central segment of a Purkinje bundle with a solution designed to mimic the extracellular milieu at a site of ischemia [107]. The gap was shown to be largely comprised of an inexcitable cable across which conduction of impulses was electrotonically mediated. Reflected reentry has been demonstrated in isolated atrial and ventricular myocardial tissues as well [109–111]. Reflection has also been demonstrated in Purkinje fibers in which a functionally inexcitable zone is created by focal depolarization of the preparation with long duration constant current pulses [112]. Reflection is also observed in isolated canine Purkinje fibers homogeneously depressed with high K+ solution as well as in branched preparations of normal Purkinje fibers [113].
Phase 2 Reentry
Another reentrant mechanism that does not depend on circus movement and can appear to be of focal origin is Phase 2 reentry [114–116]. Phase 2 reentry occurs when the dome of the action potential, most commonly epicardial, propagates from sites at which it is maintained to sites at which it is abolished, causing local reexcitation of the epicardium and the generation of a closely coupled extrasystole. Severe spatial dispersion of repolarization is needed for phase 2 reentry to occur.
Spatial Dispersion of Repolarization
Studies conducted over the past 20 years have established that ventricular myocardium is electrically heterogeneous and comprised of at least three electrophysiologically and functionally distinct cell types: epicardial, M, and endocardial cells [119, 120]. These three principal ventricular myocardial cell types differ with respect to phase 1 and phase 3 repolarization characteristics (Fig. 6.10). Ventricular epicardial and M, but not endocardial, cells generally display a prominent phase 1, because of a large 4-aminopyridine (4-AP)-sensitive transient outward current (I to), giving the action potential a spike and dome or notched configuration. These regional differences in I to, first suggested on the basis of action potential data [123], have now been directly demonstrated in a ventricular myocytes from a wide variety of species including canine [121], feline [124], guinea pig [125], swine [126], rabbit [127] and humans [128, 129]. Differences in the magnitude of the action potential notch and corresponding differences in I to have also been described between right and LV epicardium [130]. Similar interventricular differences in I to have also been described for canine ventricular M cells [131]. This distinction is thought to form the basis for why the Brugada syndrome is a right ventricular disease.
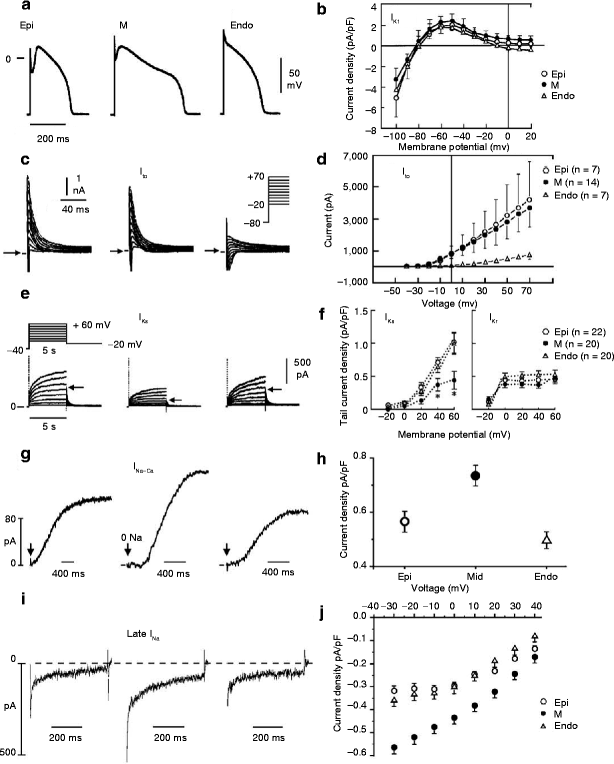
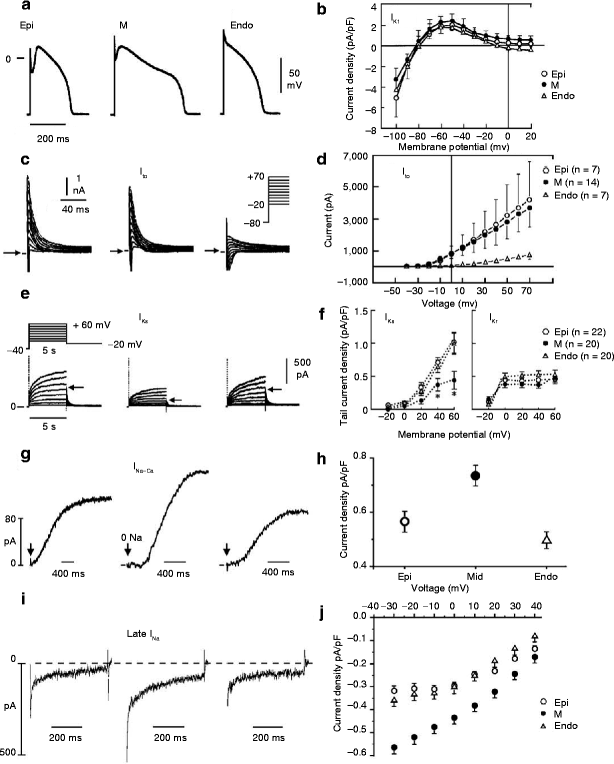
Figure 6–10.
(a) Ionic distinctions among epicardial, M and endocardial cells. Action potentials recorded from myocytes isolated from the epicardial, endocardial and M regions of the canine left ventricle. (b) I–V relations for IK1 in epicardial, endocardial and M region myocytes. Values are mean ± S.D. (c) Transient outward current (Ito) recorded from the three cell types (current traces recorded during depolarizing steps from a holding potential of −80 mV to test potentials ranging between −20 and +70 mV). (d) The average peak current–voltage relationship for Ito for each of the three cell types. Values are mean ± S.D. (e) Voltage-dependent activation of the slowly activating component of the delayed rectifier K+ current (IKs) (currents were elicited by the voltage pulse protocol shown in the inset; Na+-, K+– and Ca2+– free solution). (f) Voltage dependence of IKs (current remaining after exposure to E-4031) and IKr (E-4031-sensitive current). Values are mean ± S.E. * p < 0.05 compared with Epi or Endo (From [34, 121, 122], with permission). (g) Reverse-mode sodium-calcium exchange currents recorded in potassium- and chloride-free solutions at a voltage of −80 mV. INa-Ca was maximally activated by switching to sodium-free external solution at the time indicated by the arrow. (h) Midmyocardial sodium-calcium exchanger density is 30 % greater than endocardial density, calculated as the peak outward INa-Ca normalized by cell capacitance. Endocardial and epicardial densities were not significantly different. (i) TTX-sensitive late sodium current. Cells were held at −80 mV and briefly pulsed to −45 mV to inactivate fast sodium current before stepping to −10 mV. (J) Normalized late sodium current measured 300 ms into the test pulse was plotted as a function of test pulse potential (Modified from [122] with permission from Wolters Kluwer Health)
Myocytes isolated from the epicardial region of the left ventricular wall of the rabbit show a higher density of cAMP-activated chloride current when compared to endocardial myocytes [132]. I to2, initially ascribed to a K+ current, is now thought to be largely comprised of a calcium-activated chloride current (ICl(Ca)) which contributes to the action potential notch, but it is not known whether this current, differs among the three ventricular myocardial cell types [133].
Between the surface epicardial and endocardial layers are transitional cells and M cells. M cells are distinguished by the ability of their action potential to prolong disproportionately relative to the action potential of other ventricular myocardial cells in response to a slowing of rate and/or in response to action potential duration (APD)-prolonging agents [119, 134, 135]. In the dog, the ionic basis for these features of the M cell include the presence of a smaller slowly activating delayed rectifier current (I Ks) [34], a larger late sodium current (late I Na) [35] and a larger Na-Ca exchange current (I NCX) [122]. In the canine heart, the rapidly activating delayed rectifier (I Kr) and inward rectifier (I K1) currents are similar in the three transmural cell types. Transmural and apical-basal differences in the density of I Kr channels have been described in the ferret heart [136]. Amplification of transmural heterogeneities normally present in the early and late phases of the action potential can lead to the development of a variety of arrhythmias, including Brugada, long QT, and short QT syndromes as well as catecholaminergic VT. The genetic mutations associated with these inherited channelopathies are listed in the Table 6.1. The resulting gain or loss of function underlies the development of the arrhythmogenic substrate and triggers.
Table 6–1.
Genetic Disorders Causing Cardiac Arrhythmias In The Absence Of Structural Heart Disease (Primary Electrical Disease)
Rhythm | Inheritance | Locus | Ion channel | Gene/protein | ||
---|---|---|---|---|---|---|
LQTS | (RW) | TdP | AD | |||
LQT1 | 11p15 | IKs | KCNQ1, KvLQT1 | |||
LQT2 | 7q35 | IKr | KCNH2, HERG | |||
LQT3 | 3p21 | INa | SCN5A, Nav1.5 | |||
LQT4 | 4q25 | ANKB, ANK2 | ||||
LQT5 | 21q22 | IKs | KCNE1, minK | |||
LQT6 | 21q22 | IKr | KCNE2, MiRP1 | |||
LQT7 | (Andersen-Tawil Syndrome) | 17q23 | IK1 | KCNJ2, Kir 2.1 | ||
LQT8 | (Timothy Syndrome) | 6q8A | ICa | CACNA1C,Cav1.2 | ||
LQT9 | 3p25 | INa | CAV3, Caveolin-3 | |||
LQT10 | 11q23.3 | INa | SCN4B. Navb4 | |||
LQT11 | 7q21-q22 | IKs | AKAP9, Yotiao | |||
LQT12 | 20q11.2 | INa | SNTA1, α-1 Syntrophin | |||
LQT13 | 11q24 | I K-ACh | KCNJ5, Kir3.4 | |||
LQTS | (JLN) | TdP | AR | 11p15 | IKs | KCNQ1, KvLQT1 |
21q22 | IKs | KCNE1, minK | ||||
BrS | BrS1 | PVT | AD | 3p21 | INa | SCN5A, Nav1.5 |
BrS2 | PVT | AD | 3p24 | INa | GPD1L | |
BrS3 | PVT | AD | 12p13.3 | ICa | CACNA1C,CaV1.2 | |
BrS4 | PVT | AD | 10p12.33 | ICa | CACNB2b , Ca v β 2b | |
BrS5 | PVT | AD | 19q13.1 | INa | SCN1B, Navβ1 | |
BrS6 | PVT | AD | 11q13-14 | ICa | KCNE3. MiRP2 | |
BrS7 | PVT | AD | 11q23.3 | INa | SCN3B, Navβ3 | |
BRS8 | PVT | AD | 12p11.23 | IK-ATP | KCNJ8, Kir6.1 | |
BrS9 | PVT | AD | 7q21.11 | ICa | CACNA2D1 , Cav α2δ1 | |
BrS10 | PVT | AD | 1p13.3 | IK-ATP | KCND3, KV4.3 | |
BrS11 | PVT | AD | 17p13.1 | INa | MOG1 | |
BrS12 | PVT | AD | 3p21.2-p14.3 | INa | SLMAP | |
BrS12 | PVT | AD | 12p12.1 | IK-ATP | ABCC9, SUR2A | |
ERS | ERS1 | PVT | AD | 12p11.23 | I K-ATP | KCNJ8, Kir6.1 |
ERS2 | PVT | AD | 12p13.3 | ICa | CACNA1C,CaV1.2 | |
ERS3 | PVT | AD | 10p12.33 | ICa | CACNB2b , Cavβ2b | |
ERS4 | PVT | AD | 7q21.11 | ICa | CACNA2D1 , Cav α2δ1 | |
ERS5 | PVT | AD | 12p12.1 | IK-ATP | ABCC9, SUR2A | |
ERS6 | PVT | AD | 3p21 | INa | SCN5A, Nav1.5 | |
SQTS | SQT1 | VT/VF | AD | 7q35 | IKr | KCNH2, HERG |
SQT2 | AD | 11p15
![]() Stay updated, free articles. Join our Telegram channel![]() Full access? Get Clinical Tree![]() ![]() ![]() |