Characteristics
Pediatrics
Adults
Impact on airway resistance with reduction in airway radius (e.g., airway edema)
Greater increase
Smaller increase
Chest wall compliance
Greater compliance
Less compliance
Functional residual capacity
Lower
Higher
Respiratory muscle reserve
More reliance on diaphragm
Less reliance on diaphragm
Metabolic requirement
Higher
Lower
The Pediatric Acute Lung Injury Consensus Conference (PALICC), consisting of 27 experts from 8 countries, published recommendations for the management of PARDS after a 2-year conference process and three face-to-face meetings [8]. The panel reached strong agreement on 132 out of 151 recommendations [8]. Unfortunately, definitive data do not exist in several areas of PARDS. In those cases, recommendations were generated based on expert opinion, which may have relied on available adult or neonatal data. The lack of pediatric-specific data can be attributed to the challenges in conducting randomized controlled trials in PARDS secondary to the heterogeneity in pathophysiology, a relatively lower incidence of PARDS, and prior weaknesses in defining PARDS. Similar consensus efforts were made by The European Society for Paediatric and Neonatal Intensive Care in 2017 via the Paediatric Mechanical Ventilation Consensus Conference (PEMVECC) consisting of 15 international European experts who provided recommendations in various aspects of pediatric MV [9].
Modes of Mechanical Ventilation
A full review of all modes of conventional MV is beyond the scope of this book. In one international multicenter study, the three modes most commonly used in children with ARDS were pressure-controlled ventilation (PCV), volume-controlled ventilation (VCV), and pressure-regulated volume control (PRVC). In all three modes, the clinician programs the ventilator rate, the positive end expiratory pressure (PEEP), the fraction of inspired oxygen (FiO2), and the pressure support. The main differences between the three modes are whether the pressure or the volume is prescribed and whether the breath is sculpted with decelerating or constant gas flow. It must be noted that no mode of conventional ventilation has been demonstrated to be definitely superior in improving outcomes in pediatrics [8, 10].
Pressure-controlled ventilation – In PCV, the clinician programs the peak inspiratory pressure (PIP) and each breath is delivered over a preset inspiratory time (IT). Therefore, the tidal volume will be variable, based mainly on the respiratory mechanics of the patient. In order to quickly achieve the PIP, the flow rate is initially high, and then decreases (decelerates) to maintain the PIP for the duration of the breath. There are several potential advantages of PCV compared to VCV. Some studies show that PCV leads to higher mean airway pressures and thus improved oxygenation [11, 12]. At the same time, PIP is lower for a given tidal volume with PCV, which may reduce the risk of VILI [13]. PCV may also be more comfortable for the patient and reduce work of breathing and ventilator asynchrony [14, 15]. The main limitation of PCV is that tidal volume, and therefore minute ventilation, is not prescribed and may vary as a patient’s respiratory mechanics change. This can lead to inadequate ventilation as compliance worsens or excessive volutrauma as mechanics improve if attention is not paid to the actual tidal volume.
Volume-controlled ventilation – With VCV, the clinician programs the tidal volume on the ventilator. The ventilator uses the prescribed tidal volume and the prescribed IT to determine the correct flow to deliver. The flow is constant for the duration of the breath, sometimes termed a “square wave” flow pattern. While the tidal volume will be consistent between breaths, the PIP will vary depending on lung mechanics. The theoretical disadvantages of VCV are the opposite of the advantages listed in the above paragraph: lower mean airway pressure leading to worsened oxygenation, higher PIP leading to VILI, and reduced patient comfort. However, VCV enables the prescription of the minute volume, which may be especially beneficial when tight control of arterial carbon dioxide tension is important (e.g., intracranial hypertension, pulmonary hypertension, single ventricle physiology, etc.).
Dual-controlled ventilation (e.g., PRVC) – PRVC is intended to give the best of both worlds: the physiologic benefits of decelerating flow patterns with the clinical benefit of a prescribed minute ventilation. In PRVC, the clinician sets the tidal volume (as in VCV), but each breath is delivered with a decelerating flow pattern (as in PCV). With each breath, the ventilator adjusts the inspiratory flow rate to achieve a preset tidal volume. If the delivered tidal volume is low, the ventilator increases inspiratory pressure on the following breath. PRVC allows effective breath-by-breath tidal volume delivery while controlling PIP and adapting to changing respiratory mechanics in the patient. For many clinicians, PRVC has become the default mode for all mechanically ventilated children, including those with PARDS.
Oxygenation and Ventilation Goals
Specific oxygenation and ventilation goals may vary between patients and often within the same patient over time. The evolving pathophysiology of PARDS is patient-specific and may change in the same patient during the disease course. It is important to emphasize that clinicians need to consider the benefits of achieving optimal oxygenation against the risks of therapeutic interventions leading to pulmonary injury. Several adult and pediatric studies noted that increased systemic oxygen saturation has not been correlated with improved outcomes [7, 16, 17]. Of note, in the ARDS network low VT trial, the 12 mL/kg VT cohort had higher systemic oxygenation saturation levels than the 6 mL/kg VT cohort, but the low tidal volume group had better outcomes [7]. Furthermore, a recent trial in 434 critically ill adults showed that mortality (11.6% vs. 20.2%) and morbidity were lower in those randomized to a lower SpO2 target (94–98% vs. 97–100%) [18]. Possible explanations include immunomodulating effects of hyperoxia, free radical–induced lung injury, and higher systemic oxygen saturation requiring increased (i.e., injurious) ventilator settings. The recommended approach by PALICC was staged permissive hypoxemia depending on the severity of PARDS [19, 20]. PALICC recommended higher PEEP and lower systemic oxygenation goals with worsening ARDS [8]. In the PALICC guidelines, the PEEP recommendation for mild ARDS is <10 cm H2O, with goal systemic oxygen saturation of 92–97%. The experts further recommended that lower goal systemic oxygen saturation levels of 88–92% be considered for more severe ARDS [8]. The concept of permissive hypoxemia is attractive as it may reduce the risk for VILI, but clinicians must balance that concept with the need to provide adequate tissue oxygen delivery. Therefore, PALICC recommended monitoring central venous saturation and markers of oxygen delivery to guide setting optimal patient-specific oxygenation goals [8, 10]. In addition, the long-term impact of permissive hypoxemia has not been definitely studied. Thus, clinicians must individualize permissive hypoxemia after considering potential risks of long-term damage to end-organs as well as the potential benefits of limiting ventilator support, including FiO2. Such an approach is especially cautioned in patients who are pregnant or have pulmonary hypertension or acute intracranial pathology.
Permissive hypercapnia is recommended by PALICC in moderate-to-severe ARDS to minimize VILI [8, 10]. Adult data strongly support better ARDS outcomes with permissive hypercapnia, lower VT, and pressure-limited ventilation [21, 22]. PALICC recommended a pH range of 7.15–7.30. Similar to oxygen saturation, insufficient data exist to determine a safe lower pH limit. PALICC cautions against permissive hypercapnia in pregnant patients and those with intracranial hypertension, severe pulmonary hypertension, certain congenital heart diseases, and hemodynamically significant ventricular dysfunction. Again, clinicians should carefully assess each scenario to implement an optimal management strategy.
Tidal Volume (VT)
In the pediatric population, unlike our adult counterparts, a randomized control trial focused on tidal volume in ARDS does not exist. Pediatric providers must rely on observational data or extrapolate from adult-based recommendations of using a tidal volume (VT) of 6 mL/kg [7]. In a prospective multicenter observational study, Erickson et al. [23] studied 103 children below 16 years of age with a diagnosis of ARDS as defined by the American-European Consensus Conference. Overall mortality in this cohort was 35% during hospitalization. Interestingly, higher tidal volumes were associated with improved outcomes after adjusting for illness severity. The average maximum VT among all patients was 9.3 mL/kg (IQR: 7.8–11.6 mL/kg), and higher volumes were significantly associated with a lower odds of mortality (p = 0.03). The average median VT was 8.0 mL/kg (6.4–9.0), and higher volumes trended toward lower mortality (p = 0.08). Similar findings were observed in a retrospective single center study by Khemani et al. [24] The cohort had 198 children below 18 years of age with an overall mortality of 20%. During the first 3 days of ventilation, median VT was not significantly different between survivors and nonsurvivors. The majority received pressure-controlled ventilation (>90%) and were ventilated with a VT of 6–10 mL/kg. After controlling for diagnostic category, age, delta P (PIP-PEEP), PEEP, and severity of lung disease, VT was not associated with mortality. Interestingly, higher VT was associated with more ventilator-free days in children with less severe lung disease as assessed by dynamic compliance. In a recent meta-analysis by de Jager et al. [25], which included 8 studies with 1756 patients, mortality rates ranged from 13% to 42%. A relationship between VT and mortality was not identified when VT was dichotomized at 7, 8, 10, or 12 mL/kg. Moderate-to-substantial heterogeneity was observed in all pooled analyses. Some observational studies reported improved outcomes with higher tidal volumes of greater than 5–8 mL/kg [23, 24, 26, 27], and only one identified an association of lower mortality with VT 8 mL/kg as compared with VT 10 mL/kg [28]. In a recent prospective multicenter observational study of 23-PICUs in China, 345 infant and young children were diagnosed with PARDS as defined by American-European Consensus Conference definition. The reported mortality was 32.8% in this cohort. VT levels were not associated with mortality during the first 7 days. Furthermore, VT at levels of <6, 6–8, 8–10, and > 10 mL/kg showed no association with mortality in the first 3 days. Infants with more severe disease as indicated by higher oxygenation index (OI) and higher pediatric risk of mortality score III (PRISM III) were associated with higher mortality [27].
These observational pediatric studies seem to contradict the adult-based VT 6 mL/kg recommendation [7]. However, caution should be exercised while interpreting the observational studies. It is important to note that most pediatric patients were ventilated with pressure-limited modes of ventilation. Therefore, it could be speculated that children with more severe lung disease (i.e., poorer lung compliance) were ventilated with a lower VT than less severe counterparts. PALICC recommended using “patient-specific” VT according to disease severity. VT should be 3–6 mL/kg predicted body weight for patients with poor respiratory system compliance and closer to the physiological range of 5–8 mL/kg for patients with better preserved respiratory system compliance [8, 10]. The experts from PEMVECC had a strong agreement and recommended targeting physiological VT (5–8 mL/kg) and avoiding VT > 10 mL/kg ideal body weight [9].
It is important to note that PALICC and PEMVECC recommend using ideal/predicted body weight to determine optimal VT [9, 10]. The use of predicted weight to calculate VT is based on the assumption that volutrauma may be minimized by delivering VT appropriate to the patient’s lung capacity [7]. Martin et al. proposed unisex calculations to predict ideal body weight that can be used in pediatric patients [29]. The Center of Disease Control and Prevention (CDC) has published gender- and age-based growth charts that can be used to estimate ideal body weight (https://www.cdc.gov/growthcharts/clinical_charts.htm accessed Dec 4, 2018). Once the patient’s height/length is measured, the predicted ideal body weight corresponding to the height/length percentile can be determined easily [30].
Peak and Plateau Pressure
The ARDS Network trial demonstrated significantly lower peak and plateau pressure (25 ± 6 vs. 33 ± 8 cm of H2O, p < 0.001) in the lower VT cohort, and a linear association between mortality and peak inspiratory pressure (PIP) [7]. A recent international prospective observational study in adults (LUNG SAFE) showed higher PIP was associated with worsening from mild to moderate-severe ARDS [31]. Pediatric data are consistent with the adult literature. Studies by Khemani et al. and Erickson et al. showed a linear association between higher PIP and increased mortality [23, 24]. PALICC recommended the plateau pressure be limited to 28 cm H2O. Those experts allowed for slightly elevated plateau pressure of 29–32 cm H2O in patients with decreased lung compliance (i.e., increased chest wall elastance) [8]. In pediatrics, it should be noted that the use of variable flow ventilation and uncuffed endotracheal tubes prompts substitution of PIP with plateau pressure. In addition, an improvement in lung protection is achieved as the plateau pressure must always be the same or lower than the PIP, depending on the inspiratory airway resistance.
Positive End-Expiratory Pressure (PEEP)
Optimal PEEP is required to prevent alveolar collapse at end-expiration while avoiding overdistension, which can cause VILI and reduce right heart filling (and subsequently cardiac output). In other words, adequate PEEP titration is required to prevent atelectrauma in ARDS. In a randomized control trial of 549 adults, Brower et al. demonstrated no improvement in outcomes with higher PEEP (13.2 ± 3.5 cm H2O) as compared to lower PEEP (8.3 ± 3.2 cm H2O) when goal plateau-pressure (<30 cm H2O) and VT (6 mL/kg of predicted weight) remained within recommended ranges [32]. Similar results were found in subsequent randomized trials by Meade et al. and Mercat et al. [33, 34] Of note, these trials used PEEP/FiO2 tables as suggested by the ARDS Network study to determine higher versus lower PEEP levels but did not analyze PEEP in association with alveolar collapse [32]. Interestingly, recent meta-analyses suggest the association of higher level of PEEP with lower mortality in more severe ARDS (defined as PaO2/FiO2 ≤ 200 mmHg) [35, 36]. However, similar results were not seen in more mild ARDS. Unfortunately, there are a lack of prospective trials regarding PEEP management in PARDS.
PALICC recommended that, in absence of clear pediatric data, moderately high PEEP (10–15 cm H2O) should be titrated in severe PARDS to the observed oxygenation and hemodynamic response [8]. However, in severe PARDS, PEEP >15 cm H2O can be considered, provided peak and plateau pressure remained in the range described as above [8]. PALICC stressed the importance of monitoring markers of oxygen delivery, lung compliance, and hemodynamics with every increment or decrement of PEEP. PEMVECC had no recommendation on how much PEEP should be used. PEMVECC experts had a strong agreement that high PEEP titration is needed in severe disease by keeping a balance between hemodynamics and oxygenation. However, there is no defined method to set best PEEP [9]. Although there is no consensus regarding PEEP titration methods, previous studies have used PEEP/FiO2 titration tables recommended by ARDS network [7, 32, 34]. Interestingly, observational data in both pediatrics and adults highlight that clinicians often use lower PEEP than the levels recommended in ARDS management [8, 7, 37]. Pediatric intensivists have a tendency to use higher FiO2 over PEEP for hypoxemia during ARDS management. Several pediatric studies confirm the uncommon use of the ARDS network PEEP/FiO2 table recommendation [38–40]. In general, pediatric clinicians seem to have a reluctance to increase PEEP >10 cm H2O particularly in the younger age group [41, 38–40]. A recent multicenter retrospective trial of 1134 patients with PARDS showed that patients managed with lower PEEP relative to FiO2 compared to the ARDS network recommendations had higher mortality than children whose care aligned with ARDSnet tables [42]. In summary, there are no clear data to suggest best PEEP levels and titration method in PARDS. It is important to emphasize that markers of oxygen delivery, respiratory system compliance, and cardiovascular status should be closely monitored as PEEP is increased.
Driving Pressure
The relative importance among lower VT, lower plateau pressure, and higher PEEP is uncertain. Respiratory system compliance in ARDS is a strong determinant of volume received by the remaining functional lung. Driving pressure (∆P) is a newer concept defined as VT/respiratory system compliance (or plateau pressure minus PEEP), where VT is intrinsically normalized to functional lung size (instead of predicated lung size in healthy persons). Recent adult data (n = 3562) have shown closer association of driving pressure to ARDS mortality than PIP, PEEP, or VT alone [43]. A 1-SD increment (approximately 7 cm H2O) in driving pressure was associated with increased mortality (relative risk of 1.41, 95% CI 1.31–1.51, p < 0.001). Relative risk remained high even in patients receiving recommended plateau pressures and VT (RR 1.36, 95% CI 1.17–1.56, p < 0.001). No corresponding data or recommendations exist in the pediatric population.
Recruitment Maneuvers
Lung recruitment depends on several factors including respiratory system compliance, type of lung disease (focal versus diffuse alveolar process), and time course of lung disease. Patients with reduced lung compliance show relatively poor response to recruitment maneuvers when compared to patients with reduced chest wall compliance [44]. Limited adult data have shown an improvement in oxygenation with recruitment maneuvers in patients with preserved chest wall compliance [45]. However, a recent trial of recruitment maneuvers and titrated PEEP showed an increase in mortality among adults with ARDS [46].
With limited adult data and lack of pediatric data, significant controversy continues to exist surrounding the application and best approach regarding recruitment maneuvers. PALICC recommended the use of gradual increase or decrease in PEEP for careful recruitment. However, sustained inflation was not recommended [8, 10].
Patient-Ventilator Synchrony
Optimal patient-ventilator synchrony is of paramount importance in patients receiving MV. Achieving the optimal patient-ventilator synchrony can reduce the peak pressure and, thus, subsequently reduce the risk of VILI. Lack of synchrony between patient and mechanical ventilator can contribute to patient discomfort, dyspnea, increased energy expenditure by increasing respiratory muscle fatigue, and increased work of breathing [47]. In addition, asynchrony can lead to measurement errors in the assessment of breathing frequency and readiness to wean [48]. Difficulties in weaning may result in prolonged MV, increased ICU and hospital length of stay, increased likelihood of tracheostomy, and even increased mortality [47, 49, 50]. It is important that clinicians pay close attention to ventilator settings regardless of the mode of ventilation and titrate them according to disease evolution in a patient to optimize patient-ventilator synchrony. The goal of sedation in mechanical ventilated patients is to achieve patient comfort while maintaining safety, and it should not be used as a primary approach to prompting patient-ventilator synchrony. Excessive sedation may prolong the length of MV and increase the risk of complications in mechanically ventilated patients [51, 52]. Patient-ventilator synchrony should be achieved by close monitoring of respiratory mechanics and airway graphics, and appropriate titration of ventilator settings including inspiratory trigger and cycle time.
Summary
Unfortunately, a lack of definitive pediatric data regarding management of PARDS exists even after years of extensive research and experience. Given this lack of definitive data, variation in practice is likely to exist for individual patient scenarios as well as across clinicians and institutions. It is important to note that the clinician must frequently assess ventilator settings as the natural course of PARDS pathophysiology evolves regardless of strategy chosen. It is important to stress that the current literature does not support any ventilation mode to be superior to any other. Although PALICC has provided the pediatric community with an age-specific PARDS definition, the pediatric critical care community still must validate the proposed criteria, correlate the classification of severity with outcomes, and assess conventional as well as alternative ventilation strategies. Until definitive pediatric data become available, the majority of recommendations will continue to rely on expert opinion and extrapolation of data from adults.
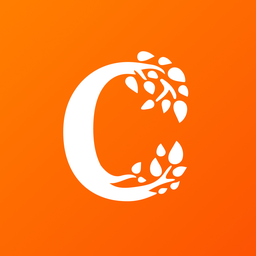
Full access? Get Clinical Tree
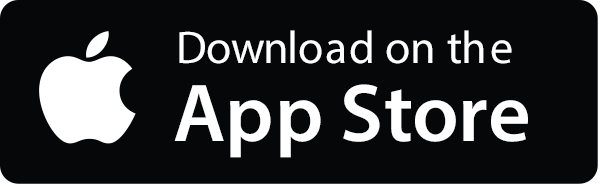
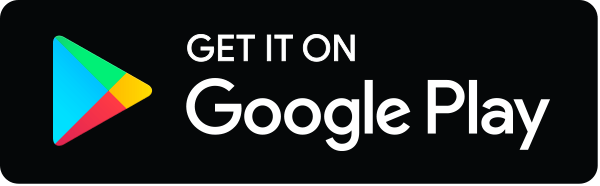