Mechanisms of gas exchange and pressure transmission during high-frequency oscillatory ventilation. Mechanisms include pendelluft (i.e., movement of gas between lung regions with different time constants), convective gas transport (i.e., transport of gas into alveoli secondary to the vacuum left after absorption of oxygen into capillaries), Taylor dispersion (i.e., passage of oxygenated gas from rapid central jet into the deeper bronchial tree), enhanced molecular diffusion near the alveolo-capillary membrane and enhanced mixing in the large airways due to turbulence. The oscillatory pressure applied at the airway opening is dampened by the resistance and inertance of the endotracheal tube and central airways. Proximal alveoli (A) are subjected to the same oscillatory pressure as the central airways, but the more distal from the airway opening, the more the oscillatory pressure is dampened, especially in compliant alveoli (C) but to a lesser extent in poorly compliant or not fully recruited alveoli (B). Increased peripheral airway resistance causes a higher pressure transmission to more proximal alveoli (E) but a lower pressure amplitude in alveoli distal of the airway resistance (F)
Clinical Evidence in Children
Summary of published pediatric randomized controlled trials or observational case-control studies evaluating the outcome effect of high-frequency oscillatory ventilation (HFOV) or airway pressure release ventilation (APRV) in critically ill children with a variable degree of pediatric acute respiratory distress syndrome (PARDS)
First author [ref] | Study design | Study period (years) | Sample size | Percentage PARDS | Main findings | Comments |
---|---|---|---|---|---|---|
High-frequency oscillatory ventilation | ||||||
Arnold [25] | RCT Five centers | 3.5 | 58 | 55 (AECC) | Survival similar between HFOV (66%) and CMV (59%) Lower need for O2 supplementation at 30 days with HFOV (21% vs. 59%, p = 0.039) | Cross-over trial, conducted in pre-ARDS network trial era Heterogeneous study population |
Samransamruajkit [26] | RCT Single center | 2 | 16 | 100 (AECC) | Survival higher with HFOV (1%), then CMV (44%) | Study not designed to examine effects on patient outcome |
Samransamruajkit [27] | RCT Single center | 1 | 18 | 100 (Berlin) | Number of deaths similar between HFOV and CMV (N = 1 in both groups) | Study not designed to examine effects on patient outcome |
Gupta [28] | Retrospective case-control 85 centers | 2 | 9177 | Unknown | In matched analysis, mortality significantly higher with HFOV (17% vs. 8%, p < 0.001) In matched analysis, mortality significantly higher with early HFO (i.e., <24 hours of intubation, 18% vs. 8%, p < 0.001) Length of MV and PICU stay significantly longer for HFOV vs. CMV | Propensity matching to overcome confounding by indication not done with variables that are commonly used in the decision-making when to go to HFOV No mention of HFOV and CMV strategy |
Bateman [29] | Post-hoc case-control | 4 | 2449 | 90 (PALICC) | Mortality rates not different between early HFOV (<24–48 hours of intubation) vs. CMV/Late HFOV in children most likely to have early HFOV (25% vs. 17%, p = 0.09) Length of MV and PICU stay significantly longer for early HFOV vs. CMV/Late HFOV | No mention of HFOV or CMV strategy Unclear how many patients really had PARDS (classification only made on OI) |
Airway pressure release ventilation | ||||||
Lalgudi Ganesen [30] | RCT Single-center | 1.5 | 52 | 100 (Berlin) | Trial stopped prematurely for increased harm with APRV (53.8% vs. 26.9% control, p = 0.089) Comparable VFDs APRV with control Comparable oxygenation APRV with control | Planned sample size was 52 per arm CMV protocol based on pediatric prone studies |
Samransamruajkit et al reported the results of a small single-center study comparing HFOV (N = 7 patients) with CMV (N = 9 patients) with AECC defined ARDS in a 2-year study period [26]. Although their study was not designed and thus powered to address this, survival was higher with HFOV (71%) compared with CMV (44%) and predicted by plasma levels of soluble intercellular adhesion molecule (sICAM) 1. The same group of investigators randomized 18 children with severe PARDS according to the Berlin Criteria to HFOV or CMV and a lung volume optimization maneuver in both groups [28]. They observed a significantly better improvement in oxygenation in patients randomized to HFOV, but again their study was underpowered to detect effects on patient outcome (in fact, only two patients died).
The controversy surrounding HFOV has been further fueled by two case-control studies. Gupta et al reported increased mortality and morbidity in patients managed with HFOV compared with CMV when they analyzed the data from the virtual PICU (vPICU) database [29]. Included in this study were 9177 patients from 98 institutions receiving MV aged 1 month to 18 years in the Virtual PICU System database from January 1, 2009, through December 31, 2011. For analytical purposes, patients were stratified by type of ventilation (HFOV vs. CMV); patients managed on the oscillator were stratified by early (i.e., within 24 hours of intubation) of and late HFOV (i.e., after 24 hours of intubation). Propensity matching to overcome confounding by indication (i.e., the sickest patient is the most likely to get the intervention) was used for matching. Comparisons between matched patients showed a significant difference in mortality (overall HFOV vs. CMV: 17% vs. 8%, p < 0.01; early HFOV vs. CMV: 18% vs. 8%, p < 0.01) and a significantly longer time spent on the ventilator for HFOV patients, challenging the use of HFOV.
Bateman and coworkers performed a post-hoc analysis of data from the Randomized Evaluation of Sedation Titration for Respiratory Failure (RESTORE) study with propensity matching to match for severity of illness comparing early HFOV (i.e., within 24–48 hours of intubation) with a group of children managed only with CMV or with CMV and late HFOV (CMV/late HFOV) [31]. For analytical purposes, they studied N = 213 children with the highest likelihood of early HFOV based on the propensity score. In-hospital mortality rates at day 90 were not different between the HFOV and CMV/late HFOV groups (25 vs. 17%, p = 0.09), but patients managed with the oscillator spend significantly more time on the ventilator in both quintiles with the highest risk of early HFOV.
Summarizing, the published pediatric clinical data are not supportive of HFOV. However, there are certain aspects that need to be considered when interpreting those data. The Arnold trial was performed during an era when the approach to CMV does not reflect current practices and the first study by Samransamruajkit et al did not report their CMV strategy [26]. Only in their second study did they mention their CMV settings, which could be appreciated as lung-protective [28]. The Gupta study is problematic to interpret, since important clinical variables that influence the decision to initiate HFOV, such as metrics of oxygenation and ventilator settings, were not available for propensity matching, thus challenging the true relevance and clinical impact of this study [32–34]. And finally, the data from the RESTORE study that was used by Bateman et al is influenced by the fact that, in that trial, decisions about MV mode and ventilator weaning strategy were left to the discretion of the treating physician [31].
Clinical Evidence in Adults
In the early 2000s, three RCTs comparing HFOV as first-line strategy with CMV in adult patients with ARDS reported improved oxygenation and – although not significant – lower mortality in patients randomized to HFOV [35–37]. A subsequent meta-analysis of all available adult and pediatric RCTs confirmed a potential mortality benefit of HFOV, although much can be said about the ventilatory management in the control groups not being compatible with what is nowadays appreciated as LPV [38]. However, continued use of HFOV in adults became highly controversial following the outcome of two large RCTs. No benefit on patient outcome was observed in the Oscillate for Acute Respiratory Distress Syndrome (OSCAR) trial that compared HFOV using a novel device with nonprotocolized CMV in 795 patients admitted to centers with little HFOV experience [39]. More worrisome however were the outcomes of the Oscillation for Acute Respiratory Distress Syndrome Treated Early (OSCILLATE). This study was stopped prematurely after inclusion of 548 out of 1200 planned patients due to increased mortality (47% vs. 35%, p = 0.005) and worse secondary outcomes in the HFOV group [40]. Unlike OSCAR, OSCILLATE was conducted in centers with previous HFOV experience and made use of a strictly protocolized CMV control arm. Including the results of these two later trials, an updated meta-analysis confirmed no outcome benefit of HFOV over CMV, challenging the routine use of HFOV in adults with moderate-to-severe ARDS [41, 42].
Why Did HFOV Fail to Improve Outcomes?
The question is whether the results of the pediatric and adult RCTs confirm that HFOV is not beneficial, or if the patient outcomes were determined by how the oscillator was used [43, 44]. Thus, one could question whether HFOV was applied in its most optimal fashion in those, in a manner that takes full advantage of the properties of HFOV. These issues (among others) include identification of the patient who will benefit the most from HFOV, the timing of cross-over from CMV to HFOV, and determining the best oscillator settings.
Indications for and Timing of HFOV
The indications for HFOV are ill-defined and are usually dictated by personal preferences of the attending physician and institutional bias. In general, HFOV is only considered as a rescue approach when CMV fails, but it could be argued that it should be considered earlier in the PARDS trajectory to minimize VILI and prevent exposure to noxious ventilator settings. There is virtually no pediatric data supporting this concept, except for one small observational study of 26 patients reporting significantly higher 30-day survival rates (58.8 vs. 12.5%) when HFOV was employed within 24 hours rather than as rescue [45]. OSCILLATE attempted to study the effects of early HFOV within 72 hours of ARDS diagnosis. However, in that study, patients could have been on the ventilator for up to 14 days prior to randomization, making it less clear what the true effects of early HFOV are [40].
Some authors have proposed the use of a specific oxygenation index (OI) or the PaO2 /FiO2 ratio as threshold in deciding when to transition a patient to HFOV. Two pediatric trials used, respectively, an OI > 13 and 15 as cut-off values [26, 27]. A recent re-evaluation of the OSCILLATE trial showed that a mortality benefit of HFOV could only be expected in adults with severe ARDS (i.e., PaO2 /FiO2 less than 100) [46], suggesting that HFOV as a rescue intervention should only be considered in the sickest of patients.
Lung Volume Optimization Maneuvers
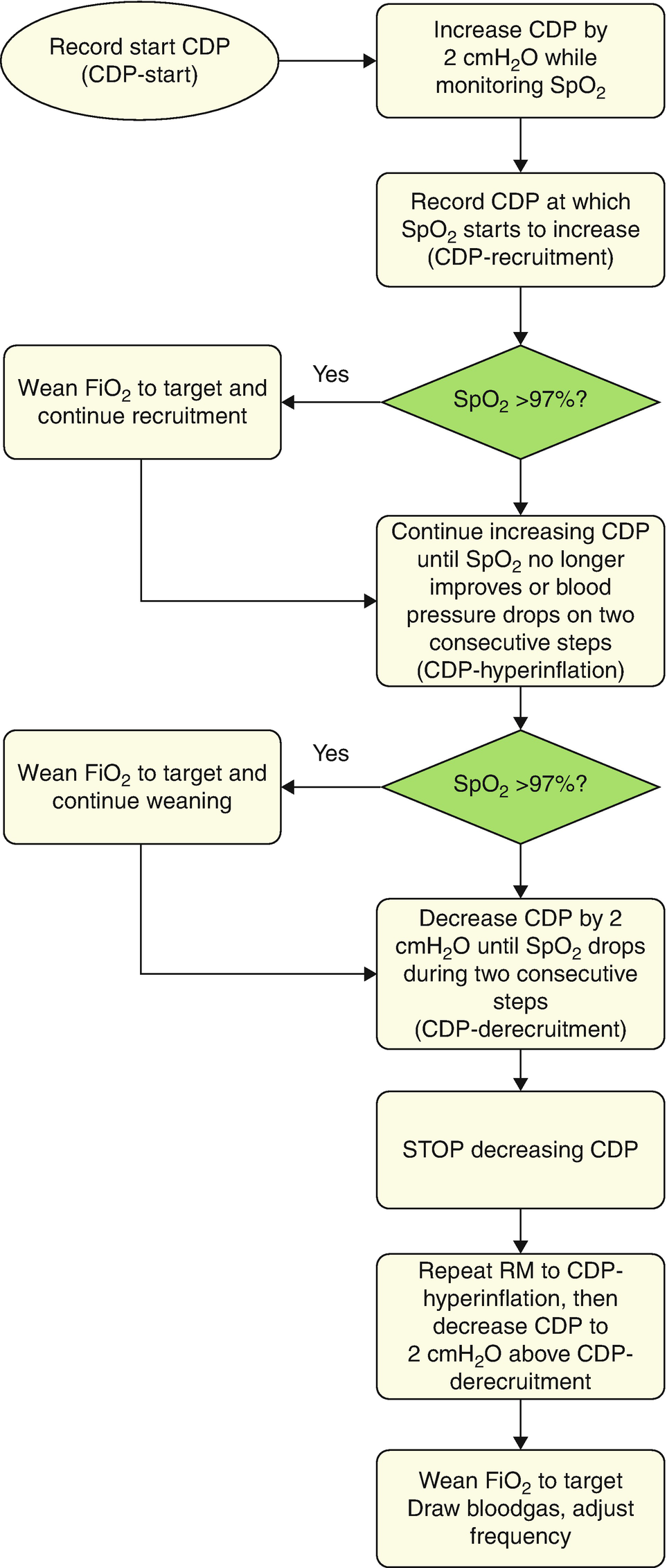
Outline of the staircase incremental–decremental titration of the continuous distending pressure (CDP) after switching to high-frequency oscillatory ventilation (HFOV)
Additional benefits of optimization of the end-expiratory lung volume include a better distal dampening of the pressure oscillations. This is because pressure oscillations are less dampened in lungs with ongoing atelectasis, thus exposing the conducting airways and alveoli to injurious higher pressure swings (Fig. 7.2) [24]. With reduced compliance in unresolved atelectasis, there is a marked increase in transmission of the peak-to-trough ∆P to the alveoli and bronchi. Another, at least theoretical, benefit of RMs is that it allows oscillating the patient on the deflation limb of the P–V curve, thereby avoiding, at least in part, injurious hyperinflation and atelectasis [20, 52–54]. By doing so, a lower CDP is needed to maintain the same amount of EELV than on the inflation limb because of the hysteresis of the respiratory system.
Achieving the Lowest Tidal Volume
The delivered Vt during HFOV is determined by numerous factors, including resistance of the respiratory system (Rrs) and oscillator settings such as oscillatory power (magnitude of membrane displacement), F in Hertz (Hz), I:E ratio, position of the membrane, endotracheal tube (ETT) length and diameter, and presence of ETT leakage [55–59]. The ETT constitutes the major work load to the oscillator and is an important determinant of Vt [60]. Vt is proportional to the ETT inner cross-sectional area because the impedance of the ETT exceeds the impedance of the lung [61, 62].
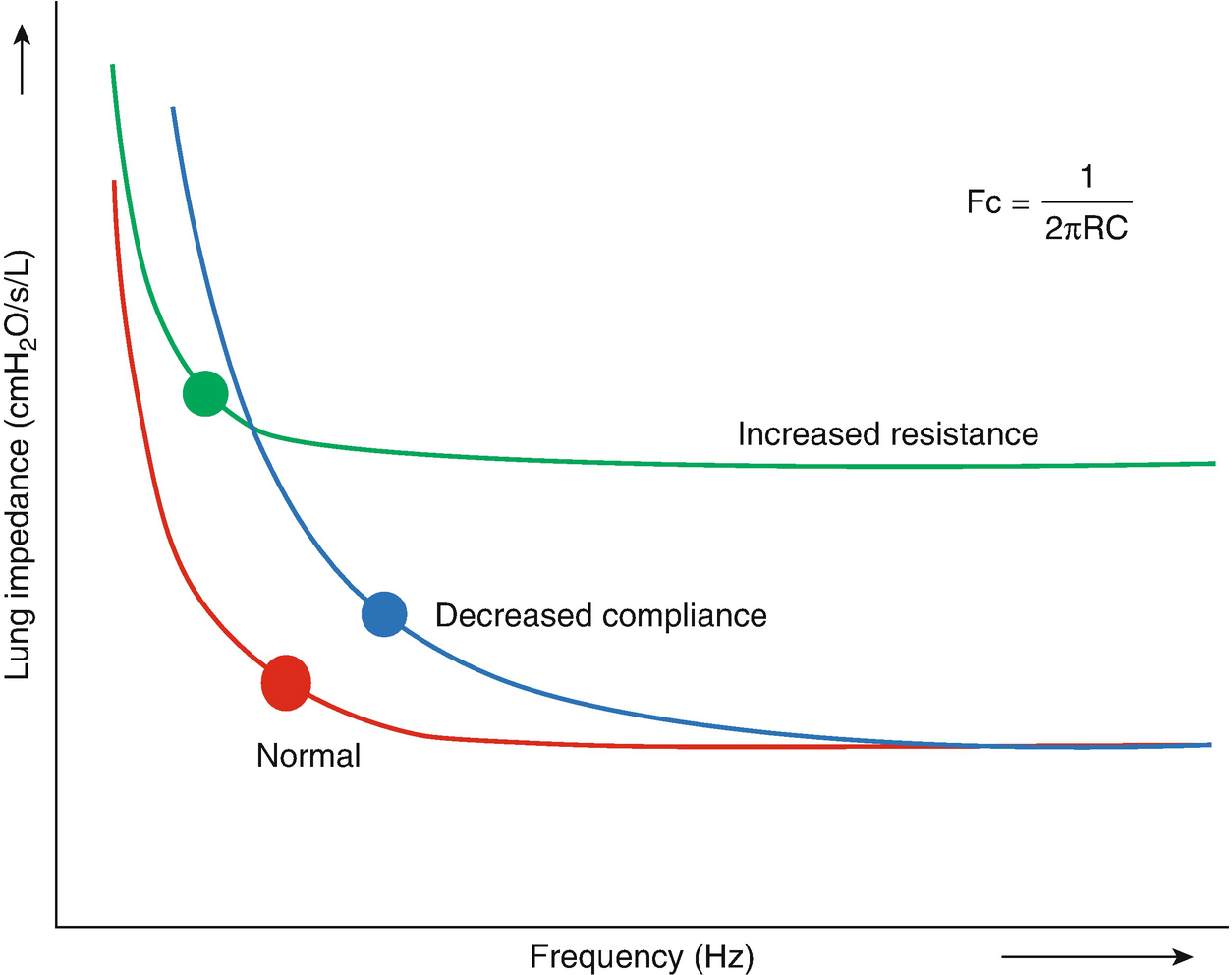
Concept of corner frequency (Fc) of the lung in patients with decreased compliance, such as PARDS and increased resistance, such as obstructive airway disease. Fc (graphically depicted by the dot) defines the optimal frequency at which there is adequate gas transport during HFOV in combination with the least injurious pressures. Fc is calculated by 1/2πRC, where R is resistance and C compliance
Airway Pressure Release Ventilation
Description of APRV
Airway Pressure Release Ventilation (APRV) was first described in 1987 as a mode to deliver two levels of continuous positive airway pressure (CPAP) while allowing for spontaneous breathing throughout the respiratory cycle [67]. Unlike traditional CPAP, both oxygenation and ventilation are supported during APRV. A continuous high flow is delivered to the patient, only interrupted by intermittent opening of the expiratory valve, resulting in a decrease in circuit pressure allowing gas outflow and thereby enhanced CO2 removal from the lungs as well as emptying of the anatomic dead space. Unlike CMV, the breath begins at an elevated baseline and ends with the deflation from high pressure to a low pressure. This driving pressure gradient establishes the delivered Vt allowing for ventilation, which is (as with PCV) affected by Crs and Rrs. Hence, APRV is considered a time-triggered, pressure-limited, and time-cycled intermittent mode of ventilation with an inversed inspiratory-to-expiratory ratio [68]. Of note, APRV is in fact an inverse ratio mode of PCV if spontaneous breathing is absent.
The operator has to set the high (Phigh) and low (Plow) pressure as well as the time (T) intervals of these pressures (Thigh and Tlow). Oxygenation is determined by the FIO2 and Thigh. Usually, Phigh is set to match the plateau pressure (Pplat) during CMV (targeting Pplat <30 cmH2O), but the approach to setting Plow and Thigh and Tlow is highly variable [68]. Thigh can be set somewhere between 4 and 10 seconds and is dictated by oxygenation. For Plow and Tlow, there are roughly two concepts: (a) using a constant Tlow and nonzero Plow to prevent complete end-expiratory lung deflation and a Thigh that is approximately 90% of the total cycle time, or (b) setting Tlow dictated by lung mechanics (to achieve an end-expiratory flow: peak expiratory flow (PEF) ratio of ±50–75%), Plow at zero and Thigh >90% of the total cycle time – the inherent short Tlow thus prevents complete end-expiratory lung emptying [69, 70]. How both are ultimately set is dictated by the desired level of CO2 elimination. The combination of these settings results in a mPaw calculated by ((Phigh ∗ Thigh) + (Plow ∗ Tlow)) / (Thigh + Tlow). The prolonged inspiratory time may lead to the development of intrinsic PEEP (PEEPi), which should be avoided because of its adverse cardiovascular effects.
Physiologic Benefits
The potential benefits of APRV are linked to a presumed reduced risk of volutrauma inherent to the pressure limitation with this mode, and therefore, the delivered Vt is affected by Crs and Rrs (as with PCV). In line with the open-lung concept, the risk for atelectrauma may be reduced because APRV delivers a higher mPaw than on PCV. Numerous animal studies have shown improved oxygenation and some reduced lung injury with APRV [30]. However, the true potential benefit of APRV is probably linked to spontaneous breathing, which makes it appealing. Critical care practitioners have adopted the philosophy of maintaining spontaneous breathing in mechanically ventilated patients as much as possible. This dogma is based on early work showing that Vt was directed toward the dorsal, well-perfused regions of the lung in anesthetized adults without lung injury when they were in the supine position [71]. These beneficial effects of spontaneous breathing were confirmed in experimental and clinical studies of lung injury [72, 73]. Explanations for these beneficial effects include reduced shunt fraction, improved distribution of Vt toward the dependent lung zones, and less lung inflammation [74–76]. In addition, spontaneous breathing may allow for a better patient–ventilator synchrony and a potential decrease in sedation and analgesia. To date, however, these beneficial effects have not been demonstrated in children.
Clinical Evidence in Children
The pediatric literature on APRV is mainly limited to case reports, case series, and retrospective and prospective observational cohort studies with small sample sizes. In most cases, APRV was employed as rescue intervention and overall results in terms of oxygenation and ventilation were not universally confirmed. In 2018, Lalgudi Ganesan et al reported the outcomes of the first RCT comparing APRV with CMV in Berlin-defined PARDS (Table 7.1) [69]. In this open-label, parallel-designed RCT, children aged 1 month to 12 years were randomized within 24 hours of PARDS diagnosis and 72 hours of ventilation to APRV or CMV. Remarkably, randomization was unbalanced as there were more girls and patients suffering from more severe hypoxia in the APRV arm. In the APRV group, Phigh was set 1–2 cmH2O above Pplat with a maximum of 30 cmH2O and subsequently titrated targeting Vt 6–7 mL/kg IBW and Thigh at 4 seconds; Plow was set at 0 cmH2O and Tlow was terminated when the expiratory flow had decreased to approximately 75% of the peak expiratory flow. Ventilator algorithms used in the two prone positioning trials by Curley et al were used for patients randomized to CMV [77, 78]. HFOV was used as rescue mode when predefined hypoxia criteria were met. Although the study was designed to include 26 patients per randomization arm, it was stopped prematurely at 50% enrollment because of increased mortality in the APRV group (53.8%) compared to CMV (26.9%), yielding a relative risk for death of 2.0 (95% CI 0.97–4.41). Cause of death in these patients was refractory hypoxemia in almost half of the patients, whereas the remaining patients died from MSOF. The primary endpoint VFDs were significantly lower in the APRV arm (9.7 ± 11.1 days) compared to the CMV arm (14.2 ± 10.4 days).
In summary, the available pediatric literature does not support the use of APRV in PARDS.
Current Evidence in Adults
Clinical studies in adults with ARDS have reported improved oxygenation, ventilation, and lung mechanics when comparing APRV with CMV, although most of these studies were not restricted to patients with severe ARDS [30]. However, different results in terms of patient outcome have been reported. Putensen et al found improved cardiovascular performance and arterial oxygenation as well as fewer ventilator and ICU days when comparing APRV with PCV, whereas others found no effect on clinically relevant outcomes in two RCTs [79, 80]. Similarly, conflicting observations have been made in prospective and retrospective cohort studies [81–83].
Why Did APRV Fail to Improve Outcomes?
Although not novel, use of APRV is limited to a certain number of centers and requires greater experience than traditional PCV. As with HFOV, there is no clear understanding of what optimal initial and titration APRV settings are effective. Furthermore, concerns related to APRV must be better understood, and include the effect of high transpulmonary pressures during spontaneous breathing, along with cyclical derecruitment and Vt variability during the release time [69].
Practice Recommendations and Future Prospects
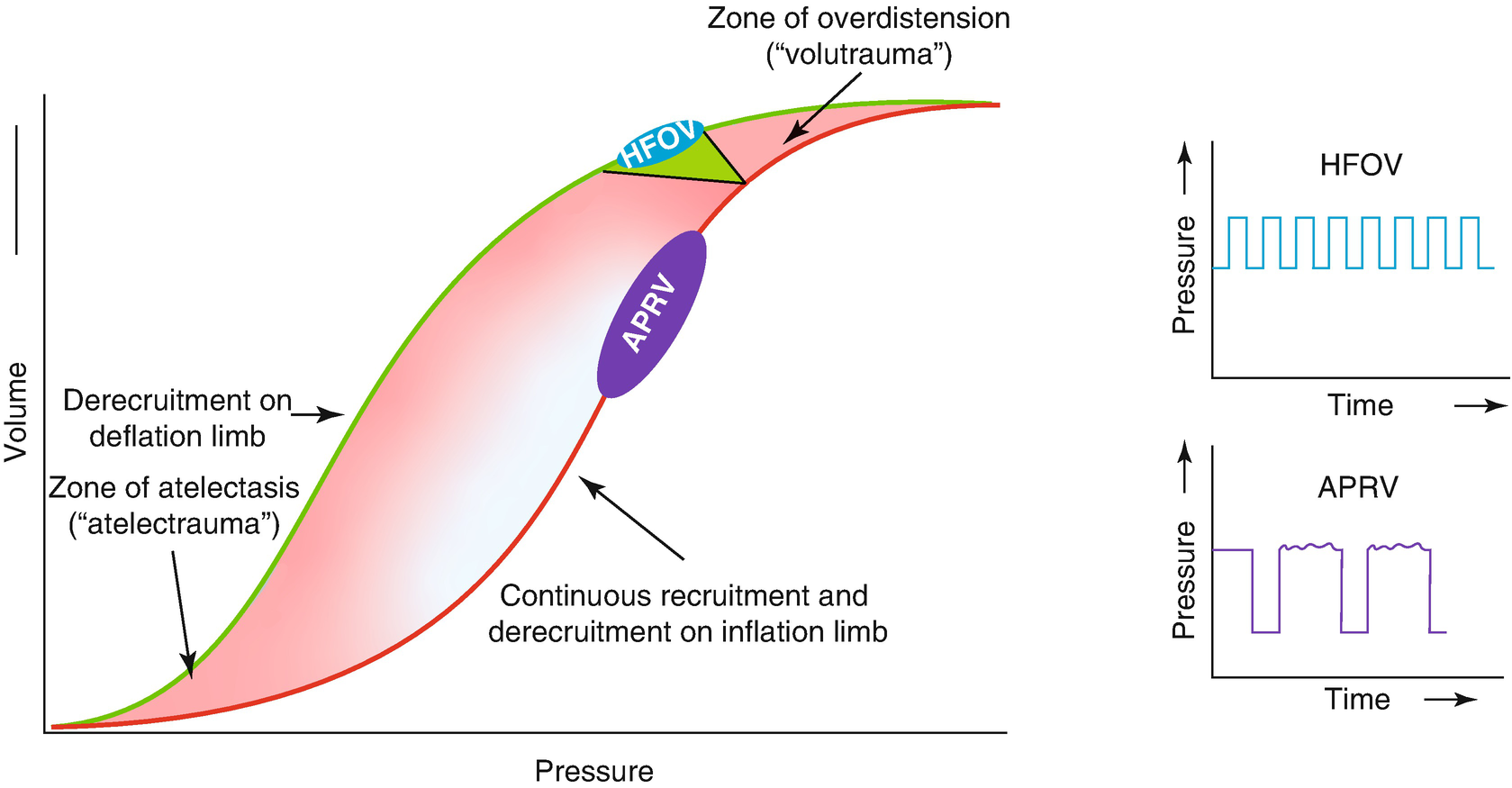
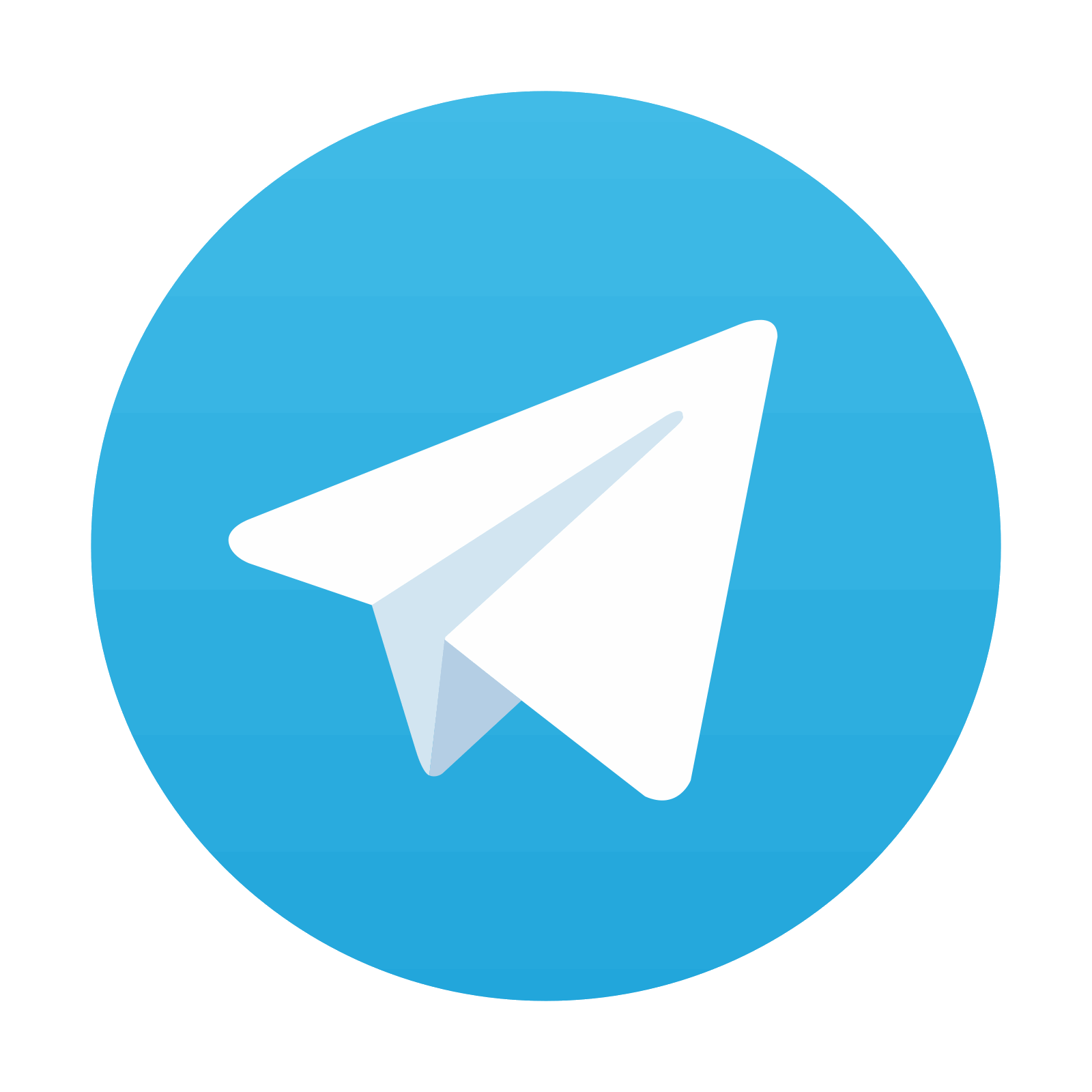
Stay updated, free articles. Join our Telegram channel
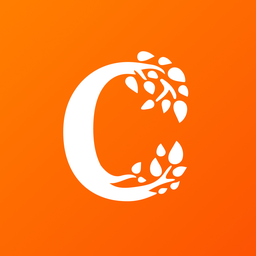
Full access? Get Clinical Tree
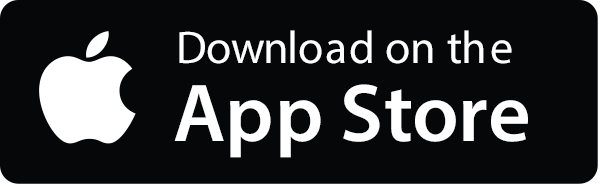
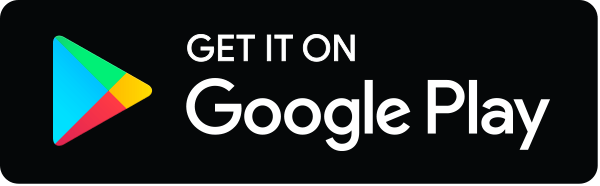