Hypertrophy
Contractile dysfunction
Calcium cycling
Cytoskeletal proteins (sarcomeric, non-sarcomeric, membrane)
Beta-adrenergic signaling
Metabolism and bioenergetics
Myocyte death (apoptosis, autophagy, stress)
Endothelium and microvasculature
Sympathetic innervation
Circulating neurohormones, cytokines
Cardiac Hypertrophy – Atrophy
Hypertrophic growth of cardiomyocytes is the common mechanism by which the heart reduces stress on the failing ventricular wall. Pulsatile LVAD unloading has been repeatedly shown to induce regression of cardiac myocyte hypertrophy – cell length, width, and thickness [10]. This is in agreement with data showing that pulsatile LVAD unloading reverses the altered cardiac production of natriuretic peptides along with parallel reductions in myocardial mass and myocyte size [4]. Regarding the exact mechanisms governing hypertrophy regression during pulsatile LVAD support, reviewed in detail elsewhere, ongoing investigations examining the complex role of Akt kinase/cyclooxygenase-2, mitogen-activated protein kinases (MAPK)/extracellular signal-related kinases (Erks), and Akt kinase/glycogen synthase kinase 3β (GSΚ3β) underscore their importance in the pathogenesis and regulation of cardiac hypertrophy. Whether the primary stimulus for the regression of hypertrophy is directly related to mechanical unloading/stretch or to circulating systemic factors needs to be further investigated. Interestingly, a study from the Harefield group examining myocytes from pulsatile LVAD patients with and without myocardial functional recovery demonstrated that myocytes from both groups had similar reductions in cell size, suggesting that hypertrophy regression might not be specifically related to the structural signature of LVAD-induced myocardial recovery [11].
Animal models of prolonged unloading in nonfailing, nonhypertrophic myocardium by means of heterotopic transplantation or LVAD or severing the chordae tendinae of the mitral papillary muscle suggested that mechanical unloading could lead to cardiac myocyte atrophy. Whether this phenomenon applies only to nonfailing and nonhypertrophic or also to hypertrophic and failing myocardium unloaded by LVAD is controversial. One animal study of hypertrophic failing hearts indicated that unloading by means of heterotopic transplantation resulted in a decrease of cardiac myocyte size beyond normal values [12]. However, in two human HF studies, unloading by means of pulsatile LVAD support also revealed a decrease of cardiac myocyte size, but not beyond the size of normal donor cardiac myocytes [13, 14]. In a later study from our group in which light microscopy findings were complemented by ultrastructural and metabolic data, we did not identify any evidence suggesting cardiac myocyte atrophy or degeneration during pulsatile LVAD support [14]. More research needs to be performed to clarify whether prolonged mechanical unloading with the currently utilized continuous flow LVADs affects basic protein degradation pathways (calcium-dependent calpain system, ubiquitin proteasome system, and lysosomal proteolysis) and/or fetal gene program overexpression, which have been implicated in cardiac hypertrophy and atrophic remodeling.
Finally, a recent study examined cardiomyocyte DNA content, nuclear morphology, and the number of nuclei per cell before and after LVAD support [15]. After unloading, the number of polyploid cardiomyocytes and cardiomyocyte DNA content declined, whereas an increase in binucleated cardiomyocytes was observed. The authors hypothesized that the vast polyploidy of cardiomyocytes in the failing human heart was a result of hypertrophic growth associated with repeated rounds of DNA synthesis that despite completion of DNA replication did not result in cell division. The increase in binucleated cardiomyocytes could suggest that reduction of noxious hypertrophic stimuli through LVAD unloading might have led to beneficial cardiomyocyte duplication and regeneration. These findings suggest that there is a dynamic and plastic regulation of cardiomyocyte content in HF, which strengthens the notion that at least a proportion of cardiomyocytes are not terminally differentiated and could reenter the cell cycle during regenerative processes. Of course, this hypothesis requires further confirmation.
Extracellular Matrix
Remodeling of extracellular matrix, specifically an increase in fibrosis, is a hallmark feature of myocardial remodeling in chronic HF. Altered collagen metabolism is responsible for ventricular dilatation and for changes in systolic and diastolic function. Investigations of the effect of mechanical unloading on extracellular matrix have shown conflicting results: one group of investigators has reported decreased fibrosis, while others found an increase in fibrosis associated with an increase in cross-linked collagen and myocardial stiffness [2, 3, 10]. The explanation for the contradictory observations is not clear, with some attributing the inconsistent results to differences in the methodology employed [2, 3]. Applying recent advances in whole-field digital microscopy, we addressed this issue using digital histopathology and advanced image analysis techniques, an approach that reduces observer bias, increases the amount of myocardial tissue analyzed, and permits comprehensive endocardium-to-epicardium evaluation [14]. This eliminates the confounding effect of endocardial or epicardial sampling known to be associated with different degrees of fibrosis. In failing hearts, interstitial and total fibrosis was higher compared with normal myocardium, and the collagen content increased further after LVAD unloading [14].
Changes in the neurohormonal milieu seen after LVAD implant support the above findings. While older studies indicated that circulating levels of many neurohormones (plasma epinephrine, norepinephrine, arginine, vasopressin, renin, and angiotensin II) decrease after LVAD implant, the effects of LVAD unloading on the myocardial tissue renin-angiotensin-aldosterone system (RAAS) components (including the profibrotic and prohypertrophic angiotensin II) seem to be more complicated. Klotz et al. recently published results of the first study that systematically analyzed the different components of RAAS in paired myocardial tissue samples obtained before and after LVAD implantation [16]. Renin levels in the pre-LVAD myocardium were the highest ever reported in human cardiac tissue (100× normal), and the myocardial aldosterone level was also elevated (250× normal). After LVAD support, myocardial renin and aldosterone levels markedly decreased, but, in contrast with this finding, myocardial angiotensin I and II levels increased five to ten fold [16]. The increase in the myocardial level of angiotensin II during LVAD support was accompanied by a seven fold rise in myocardial norepinephrine content [16]. Increased norepinephrine levels are also known to lead to cardiac fibrosis. The same group of investigators reported an increase in the ratio of matrix metalloproteinases (MMPs) to tissue inhibitors of metalloproteinases (TIMP-1) in end-stage HF, which normalized after LVAD unloading, favoring decreased collagen degradation and hence increased fibrosis [17]. Collectively, these post-LVAD myocardial biomarker alterations are compatible with the structural findings, suggesting increased post-LVAD fibrosis. Whether the observed increase in fibrosis is a manifestation of further progression of cardiac remodeling that LVAD unloading failed to reverse or a direct result of LVAD actively inducing an increase in fibrosis warrants further investigation.
Endothelium and Microvasculature
Myocardial microvascular density is reduced in patients with HF. LVAD unloading has been shown to lead to changes in the expression of genes involved in the regulation of vascular organization and migration. In agreement with these findings, our group demonstrated that pulsatile LVAD unloading resulted in increased microvascular density in failing human hearts [14]. We also found strong evidence of endothelial cell activation by both immunohistochemistry (the endothelial activation marker major histocompatibility complex class-II, MHC-II) and by electron microscopy (ultrastructure analysis) [14]. The findings of a post-LVAD increase in microvascular density and endothelial cell activation were also accompanied by increased interstitial and total myocardial fibrosis [14]. This suggests that the recently described mechanistic link between the endothelium and cardiac fibrosis during the cardiac remodeling process – “endothelial to mesenchymal transition” via pathways directly implicated in cardiomyocyte hypertrophy [18, 19] – might also apply to human myocardium. Obviously, direct proof for such a mechanism requires lineage tracing possible only in genetically manipulated animal models [18, 19]. Of note, work done in our laboratory has shown that endothelial proliferation and migration, hallmarks of angiogenesis, must be balanced by mechanisms that stabilize the endothelium so that a functional vascular network may be established and maintained [20–22]. An imbalance in these competing signals after LVAD implantation may contribute to the increase in microvascular density, endothelial activation, and cardiac fibrosis.
Beta-Adrenergic Signal Transduction
The changes in the β-adrenergic receptor (AR) system in the failing heart are well known. Typically, the β1-AR density is selectively reduced, and the remaining β1- and β2-AR are desensitized. The response of cardiomyocytes to β-adrenergic stimulation with isoproterenol after long-term LVAD support was characterized by an increase in the magnitude of contraction and shortening of the time to peak contraction and time to 50 % relaxation [23]. In isolated trabecular muscles of failing human hearts with and without LVAD support, Ogletree-Hughes et al. found that unloading restores the density of β-AR in cardiomyocytes [24]. They also found that muscle from hearts that had been supported with LVAD produced an inotropic response to isoproterenol similar to non-failing hearts, significantly stronger than that observed with muscles from failing hearts that had not been mechanically supported. However, the receptor density alone did not predict the magnitude of the inotropic response [24]. Similarly, in another study, an increase in β-adrenergic density and a prominent normalization in the location of the receptors in the myocardium were observed following ventricular unloading [25]. The potentially important role of β-AR signaling in the reverse remodeling process that occurs in mechanically assisted failing hearts is also supported by experimental observations showing that β2-AR stimulation results in inotropic support of the heart and decreases apoptosis, while β1-AR causes progressive cardiomyopathy and HF.
Altogether, these observations suggest that it might be beneficial to combine ventricular unloading with an augmentation of β2-AR signaling to enhance reverse remodeling. In this direction, in order to maximize the efficiency of LVAD as a bridge to recovery, M.H. Yacoub combined mechanical unloading with the selective β2 agonist clenbuterol to take advantage of the therapeutic effects of β2-AR signaling on the heart as well as of the antiatrophic effects of clenbuterol on the mechanically unloaded myocardium and on skeletal muscles [26]. According to the Harefield protocol, clenbuterol is added to standard medical treatment for HF, including β1-blockade, angiotensin-converting enzyme, or angiotensin-II inhibition, spironolactone and digoxin, when maximal regression of the LV end-diastolic and end-systolic diameters has been reached, usually after approximately 2 months of LVAD support, and when they have remained stable for at least 2 weeks. Using this strategy, Yacoub’s group was able to remove LVAD successfully from 11 out of 15 patients suffering from non-ischemic cardiomyopathy, and excellent cardiac function was preserved during long-term follow-up [7].
Whether stimulation of β2-AR with clenbuterol best takes advantage of the beneficial effects of β2-AR signaling described earlier in order to enhance the effectiveness of chronic mechanical unloading, with myocardial recovery as a target, certainly needs further investigation.
Effects of LVAD-Induced Unloading on Myocardial Function
Ventricular remodeling is associated with a rightward shift of the pressure-volume loop toward larger volumes, while pulsatile LVAD unloading results in a leftward shift in the direction of a normal physiological relationship. Both continuous flow and pulsatile flow LVADs are associated with significant volume unloading and subsequent decrease in LV end-diastolic diameter. However, pulsatile LVADs seem to have a more pronounced volume unloading effect compared with continuous flow LVADs [10]. Continuous flow LVADs, in addition to LV unloading, have also been associated with significant left atrial volume unloading and improved left atrial function at 3–6 months after LVAD implantation [10].
LVAD unloading results in increased cardiac output and near normalization of pulmonary artery pressures and LV systolic and diastolic pressures. These changes are comparable regardless of the etiology of HF and are similar in magnitude in pulsatile and continuous flow LVADs. Etz et al. reported that continuous flow LVADs implanted in patients with medically refractory pulmonary hypertension resulted in a significant reduction in mean pulmonary artery pressures and pulmonary vascular resistance, and these patients were subsequently successfully transplanted [27].
Left ventricular unloading by LVAD also has significant effects on the right ventricle (RV). LVAD unloading will typically result in a decrease in RV afterload and improved RV geometry and systolic function [4]. However, higher than optimal continuous flow LVAD unloading can result in a leftward shift of the interventricular septum with a resulting compromise in RV systolic function and RV failure [4].
Exercise intolerance in patients with chronic HF is associated with respiratory muscle weakness and poor outcomes [10]. In a study where cardiopulmonary exercise testing was done before and after continuous flow LVAD placement, we observed that all patients had a restrictive ventilatory pattern before LVAD implantation. This improved during LVAD support and was associated with a significant increase in the anaerobic threshold, peak work rate, and exercise duration, findings consistent with other investigations [10].
Experience from “Bridge to Recovery Studies”
The main results of key clinical outcome studies investigating LVAD used as a bridge to recovery are summarized in Table 49.2 [4–8, 28–33]. Most of the clinical outcome studies that addressed myocardial recovery during LVAD support were retrospective, and the results, as far as the success of LVAD weaning and of achieving sustained myocardial recovery, varied significantly (Table 49.2). These inconsistencies can be explained by numerous limitations in the study design, such as (1) absence of a pre-specified protocol to monitor functional myocardial recovery, (2) absence of a pre-specified protocol for the use of adjuvant pharmacotherapy with potential anti-remodeling effects, (3) variable duration of LVAD support, (4) lack of standardized LVAD explantation criteria, and (5) diversity of the populations studied in their propensity for recovery. With the exception of three recent studies from Berlin, Harefield, and Vancouver [8, 28, 34], the majority of the devices utilized in the bridge to recovery studies have so far included first generation, pulsatile LVADs. The most effective approach aimed at recovery of myocardial function reported so far is that of the Harefield protocol, which tested mechanical unloading combined with aggressive anti-remodeling drug therapy, including the beta-2 agonist clenbuterol, in advanced non-ischemic cardiomyopathy patients [4, 7, 8]. Reproducibility of these results in larger patient cohorts and in a randomized fashion would be of great importance.
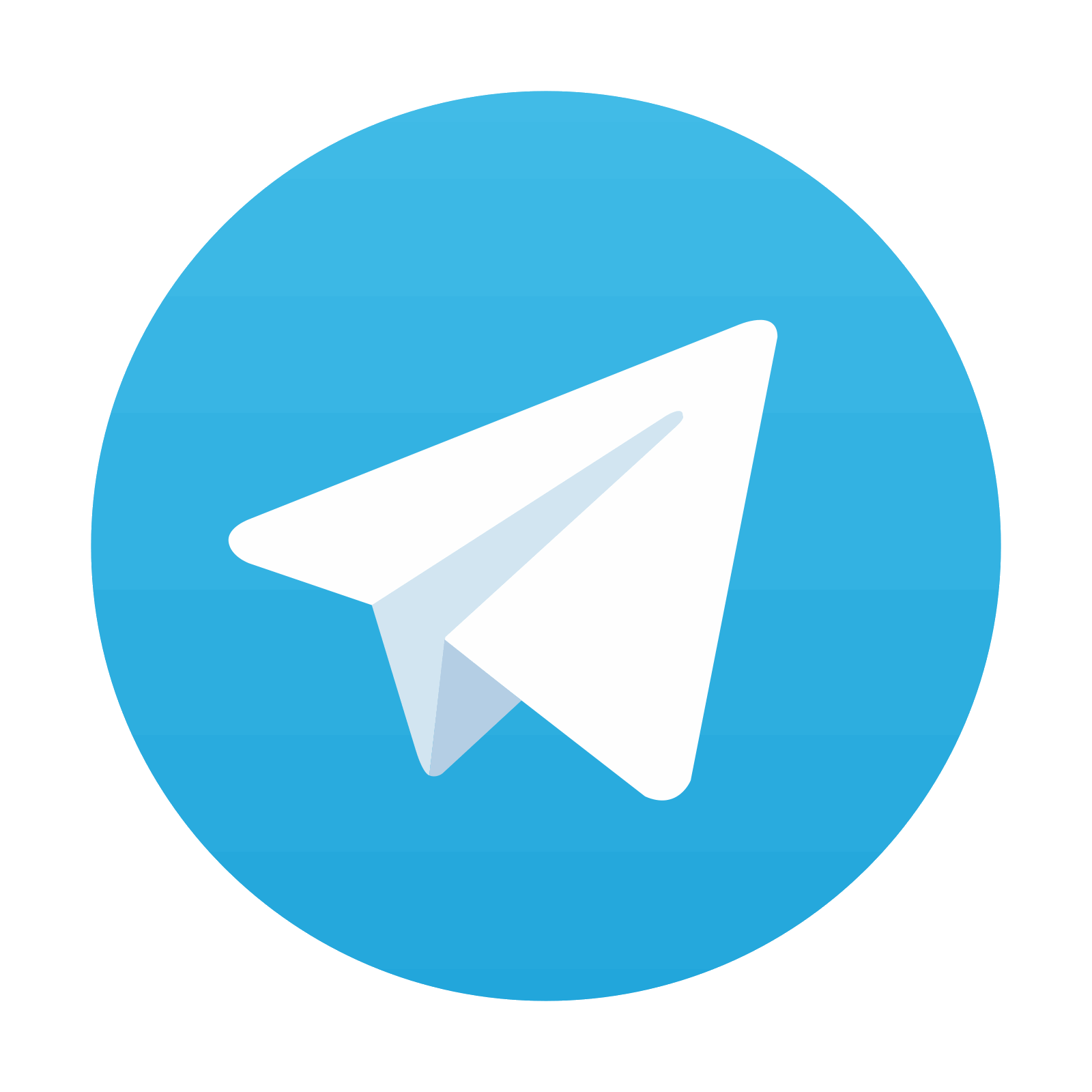
Table 49.2
LVAD bridge to recovery studies
< div class='tao-gold-member'>
Only gold members can continue reading. Log In or Register a > to continue
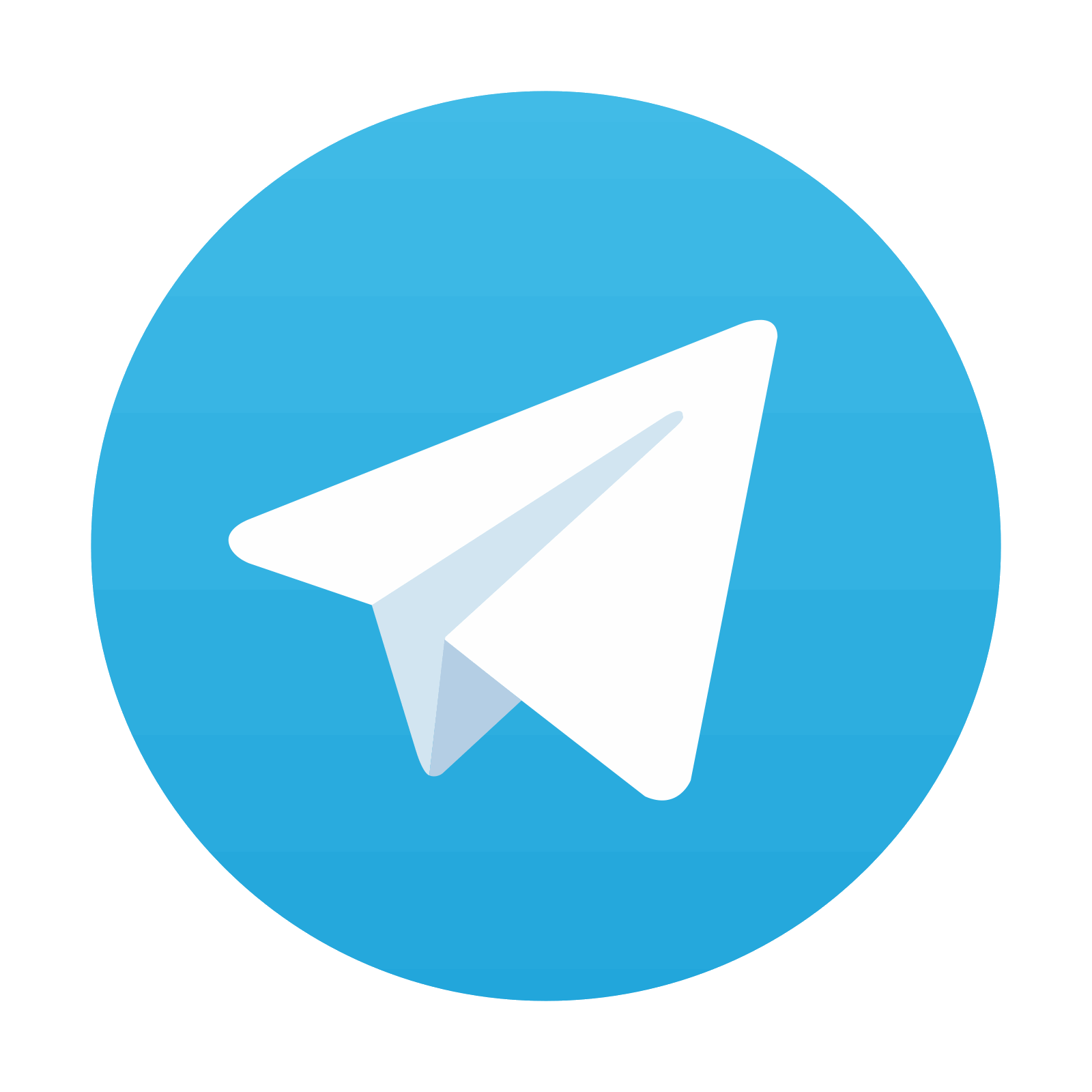
Stay updated, free articles. Join our Telegram channel
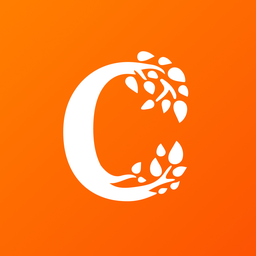
Full access? Get Clinical Tree
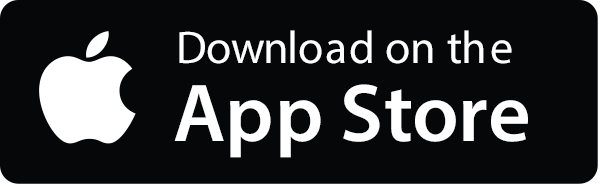
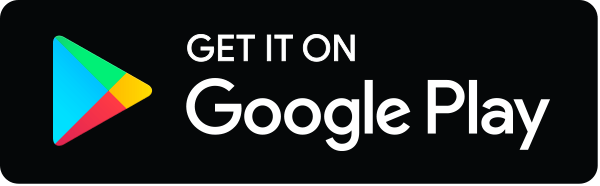
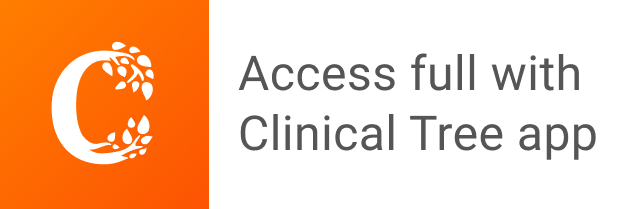