Preexisting RV dysfunction
Congenital disease
Pulmonary arterial hypertension
Coronary artery disease
Valvular disease
RV myocardial infarction
Coronary embolism
Thrombotic occlusion
Graft occlusion
Suboptimal myocardial protection
Prolonged CPB time
Postoperative pulmonary arterial hypertension
Lung ischemia-reperfusion injury
Protamine administration
Excessive blood transfusions
Pulmonary embolism
Excessive volume loading
Volume infusion
Multiple blood transfusions
Severe tricuspid regurgitation
Unloading of the LV
LVAD implantation
Pericardial constriction
Postcardiotomy syndrome
Sepsis
The pathophysiology of postcardiotomy ARVF is described schematically in Fig. 12.1. It is mainly precipitated by an element of ischemia. During cardiopulmonary bypass (CPB), pulmonary vascular resistance (PVR) may increase because of complement activation, hemodilution, and autologous blood transfusion [11]. When the RV fails acutely, maintenance of hemodynamic status depends on the contractile state of the LV and the interventricular septum together with right atrial contractility and atrioventricular synchronization [12, 13]. The role of the interventricular septum should be emphasized, as LV septal contraction into the RV can compensate by providing pulmonary perfusion [14]. Most patients only develop a mild degree of RV dysfunction as a well-functioning LV is capable of maintaining a satisfactory hemodynamic status, provided there is no significant element of PAH. When normal compensatory mechanisms fail to preserve hemodynamic stability, the RV dilates passively due to volume loading and the RV end-diastolic volume is increased. LV contraction against a passively dilated RV leads to tricuspid regurgitation. RV dilation causes a leftward shift of the interventricular septum, increases pericardial constraint, and modifies acutely LV geometry (ventricular interdependence, analyzed in Pathophysiology chapter). As a consequence, both LV distensibility and contractility may be decreased resulting in reduced cardiac output [15]. ARVF leads to systemic congestion and circulatory failure. Hypoxemia is often noted that may occur as a consequence of increased right to left shunting through a patent foramen ovale or increased ventilation-perfusion mismatch associated with reduced cardiac output.
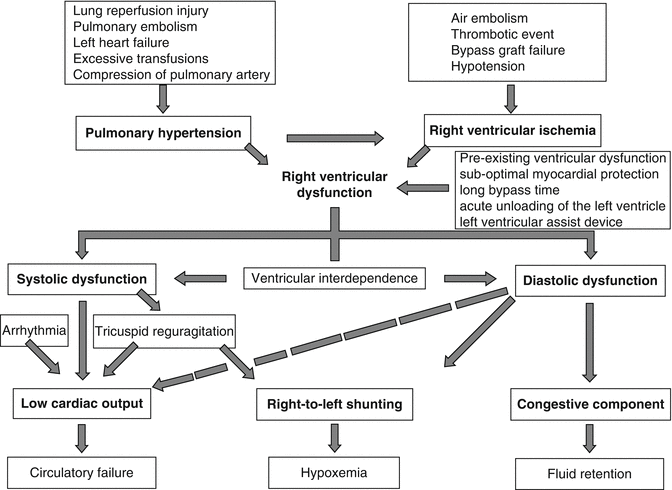
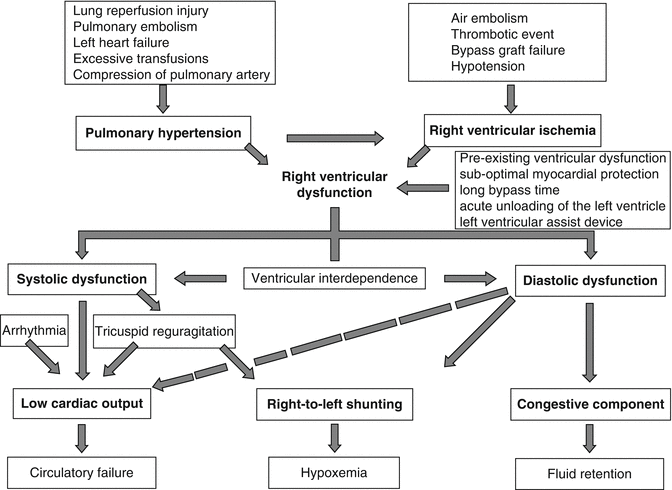
Fig. 12.1
Pathophysiology of postcardiotomy refractory ARVF
The management algorithm of AVRF is configured in Fig. 12.2. Patients with persistent ARVF that fail to improve despite high-dose inotropic/vasopressor support (as discusses in Pharmacology chapter) and optimal medical management represent a high-risk group that requires temporary mechanical circulatory support (MCS) of the RV as a life-saving procedure, in order to preserve end-organ perfusion and reverse the cascade of multisystem organ failure. The RV exhibits a greater capacity for rapid recovery compared with the LV. In a significant proportion of patients (42–75 %), RV recovers sufficient function that allows discontinuation of MCS [16, 17]. Different types of MCS have been used to assist the RV. The ideal device designed for the treatment of potentially recoverable ARVF should be an easily deployable, minimally invasive RV assist device (RVAD) that could completely off-load the RV. In patients with acute RV failure refractory to medical treatment, mechanical support with an RV assist device may be used as a bridge to transplantation or to recovery. It should be noted that RVADs have successfully assisted in bridging to transplant or recovery in patients with RVF following cardiotomy with evidence of improved hemodynamic stability. However, the small number of patients and varying indications for an RVAD seen in the studies combined with the high mortality and morbidity rates associated with RVADs suggests that there is no clear evidence of the benefit for his strategy [18].
12.1.2 Intra-aortic Balloon Pump
Given the ease of percutaneous implantation, acceptable cost, and its low complication rate, the intra-aortic balloon pump (IABP) stands as the more convenient and first applicable form of mechanical assistance available in most cardiac and general intensive care units. It is easily implanted through the femoral artery in the descending thoracic aorta of patients with postcardiotomy cardiogenic shock and it assists the LV by reducing end-diastolic pressure and LV afterload. Other beneficial effects are a decrease in LV volume, systolic work, and myocardial oxygen consumption together with reduced end-diastolic and peak systolic aortic pressure. Use of IABP can improve LV output by 20 % [19, 20]. The influence of the IABP on ischemic RV function is much debated. RV dysfunction complicates around half of inferior-wall infarctions and occurs to some degree in 20–30 % of patients who receive an LVAD [21]. A porcine model of acute ischemic right heart failure with cardiogenic shock, constructed by Nordhaug and colleagues, showed that use of an IABP had no influence on right or left ventricular contractility [22]; however, it resulted in significantly improved cardiac output, lower PVR and increased RV efficiency, as measured by stroke work per pressure-volume area compared with controls. Thus, it offers an indirect beneficial effect on RV function derived mainly from reduced pulmonary arterial resistance.
In the early 1970s, experimental and clinical studies evaluated the use of balloon pump in the pulmonary artery (PA) as a temporary RVAD. They have demonstrated that a PA balloon pump reduces pulmonary arterial systolic pressure and off-loads the RV [23–25]. Eventually, RV performance improves and cardiac output is restored to satisfactory levels. The PA balloon pump support has been used, but this was suitable for only a short-time period in patients with up to a 50 % reduction in optimal RV performance. It is unsuitable for extended use and not as reliable as the RVAD support [26]. For these reasons, use of the PA balloon pump support has been abandoned in favor of RVAD systems.
12.1.3 Extracorporeal Life Support (ECLS)
Extracorporeal life support (ECLS), commonly referred to as extracorporeal membrane oxygenation (ECMO), is used to sustain physiological levels of blood flow in cardiogenic shock and more recently as a rescue system during cardiopulmonary resuscitation [27, 28]. The main advantage of ECLS over other forms of MCS is that it provides both cardiac and pulmonary support for patients with cardiac and respiratory failure who are usually in profound hypoxia. An ECLS circuit consists of a centrifugal blood pump, membrane oxygenator, and a heparin-coated circuit (Fig. 12.3). It represents the simplest and most rapid method of restoring systemic blood flow and, with contemporary peripheral percutaneous arteriovenous cannulation techniques, can now be used in the catheterization laboratory or emergency room [29]. The circuit takes around 10–15 min for the perfusionist to assemble. Cannulation of the femoral artery and vein are achieved rapidly by the Seldinger technique, using specifically designed perfusion cannulae over a guidewire, even during cardiac resuscitation. The tip of the femoral arterial cannula is advanced to the aortoiliac junction and the venous drainage cannula advanced into the lower right atrium. In postcardiotomy ARVF, open surgical cannulation of the ascending aorta and the right atrium can be performed. Central cannulation provides higher flows but requires surgical decannulation and predisposes to infectious and bleeding complications. Minimal systemic heparinization is used so as to achieve an activated clotting time of 160–180 s.
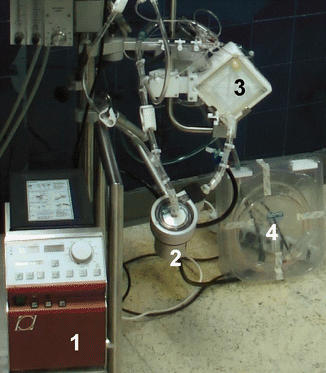
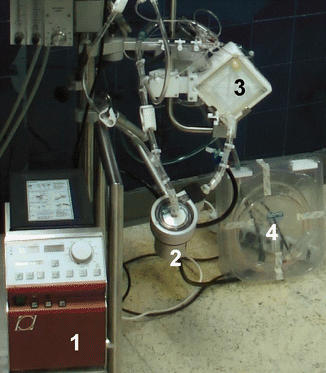
Fig. 12.3
The ECLS circuit. Note the simplicity of the system which comprises of a controller (1), centrifugal pump (2), oxygenator (3), heparinized tubing (4)
Once the circuit is established, the flow rate can be progressively increased to 3.5–4.0 l/min, thus almost fully off-loading/decompressing the RV and restoring a normal cardiac index. The mean blood pressure is maintained at 75–85 mmHg. A pulsatile arterial pressure trace confirms residual myocardial contractility and reduced risk of intracardiac clot formation. If pulsation disappears, volume expansion or inotropic support is used until pulsatility is restored. Rapid stabilization of hemodynamic status during ECLS enables biochemical derangement to be corrected and allows time to determine cerebral status following cardiac arrest. The potential for myocardial recovery, cardiac transplantation, or a long-term LVAD use can then be assessed (Fig. 12.4).
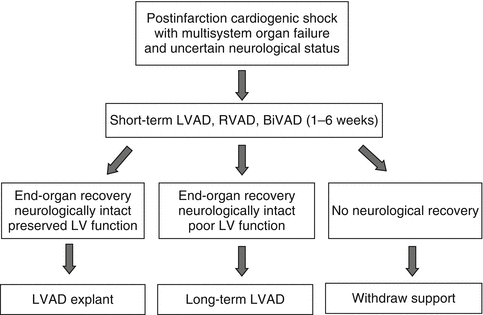
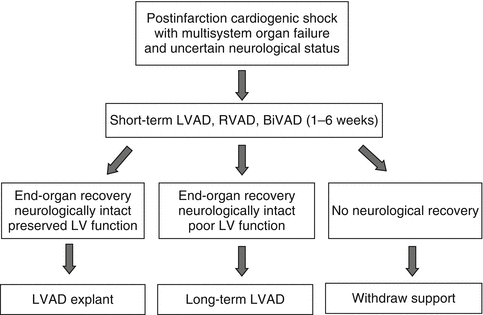
Fig. 12.4
Decision algorithm for patients with refractory ARVF
Drainage of blood from the circulation into the ECLS circuit results in decreased right atrial and central venous pressure (CVP). This results in decreased RV ejection into the lungs and decreased pulmonary venous return to the left atrium, thus reducing LV end-diastolic volume and pressure. This unloading of the RV and LV reduces myocardial oxygen demand and promotes myocardial recovery. Return of blood into the aorta can lead to improved end-organ and coronary perfusion. Animal experiments have shown that decreased RV and LV preload during ECLS are associated with a transient decrease in contractility of both ventricles, which is not related to impaired intrinsic myocardial contractile function [30]. This load-dependent decrease in contractile function is usually transient and recovers within 72 h after onset of ECLS support. As previously noted, decrease in end-diastolic volume and pressure decrease wall stress, improve coronary perfusion, and decrease workload posed to the ventricle, thereby promoting myocardial performance and contractility. Interpretation of myocardial contractility in patients supported with ECLS should always take into account preload conditions at which time examination is performed, keeping in mind that contractility may alter in different filling conditions making assessment of cardiac function error prone [31]. Recovery of contractile function and arterial pulsation is a positive sign of myocardial recovery, and the timing of such recovery has been associated with survival [32]. Failure of return of ventricular function within 72 h of the institution of ECLS support is an ominous sign related to increased mortality [31].
It should be noted that support with venoarterial ECLS provides optimal RV unloading and respiratory support. However, LV afterload and wall stress is increased due to vasoconstriction from compensatory sympathetic activation triggered by decreased cardiac output prior to onset of ECLS. Increased mean arterial pressure caused by retrograde blood flow into the aorta contributes to this effect. In cases where the systolic pressure generated by the impaired LV is inadequate to open the aortic valve, loss of native ejection occurs, arterial pulsatility decreases, and LV end-diastolic volume and wall tension increase. Moreover, blood volume return from bronchial circulation and thebesian vessels directly into the left atrium further increases LV volume. Subsequent left atrial hypertension (left atrial pressure >20 mmHg) can cause pulmonary edema and impair gas exchange to the lungs related to a dismal prognosis. This mandates prompt institution of some form of LV decompression. The LV can be vented either by mini-thoracotomy and direct cannulation of the left atrium or LV or percutaneously through a transseptal atriotomy creating a small atrial septal defect in the catheterization laboratory [33]. A minimally invasive technique has also been proposed using the pulsatile paracorporeal assist device iVAC 3 L (PulseCath, Groningen, the Netherlands) through the right axillary artery [34]. Thus, it is critical to maintain cardiac contractility in order to avoid LV distension, clot on the akinetic myocardium, or development of PAH with judicious use of inotropes or IABP support during ECLS.
In conclusion, ECLS provides effective RV off-loading and respiratory support and can be used either in the catheterization laboratory or in the operating room as a bridge to recovery (such as post-acute myocardial infarction, pulmonary embolism, or myocarditis), bridge to decision, or bridge to long-term MCS.
12.1.4 Percutaneously Implanted Blood Pumps
Potentially recoverable ARVF not related to cardiotomy, such as after massive pulmonary embolism or RV infarction, highlights the need for easily deployable, minimally invasive RVADs that could be inserted percutaneously in the catheterization laboratory and provide unloading of the acutely failing RV. The available devices designed specifically to provide temporary support for ARVF used in the clinical practice are mainly Impella RP (Abiomed Inc, Danvers, MA, USA) and TandemHeart-right ventricular support device (CardiacAssist, Inc., Pittsburgh, PA, USA).
The Impella CP, which is inserted percutaneously and provides up to 2.5 l/min of support together with surgically implanted Impella 5.0, is a popular device for ventricular support which is widely used for both cardiology and cardiac surgery patients. The surgically implanted Impella 5.0 has two modifications. The LP designed for LV support and the RP, which is specifically designed for the RV. Impella RP is a three-dimensional catheter-based microaxial pump designed specifically for temporary RV support. The device consists of a 23 Fr pump head containing the electric motor, axial blood pump, and outflow cannula, mounted on an 11 Fr catheter. It is placed percutaneously through the femoral vein and advanced in an antegrade fashion across the pulmonary valve into the PA under transesophageal echocardiographic and fluoroscopic guidance. The inflow portion of the catheter resides in the inferior vena cava, and a flexible nitinol cannula traverses the right atrium, tricuspid valve, and pulmonary valve. The outflow portion of the catheter resides in the main PA (Fig. 12.5). Anticoagulation with heparin is initiated to maintain a partial thromboplastin time of 45–80 s. The Impella RP can provide flow up to 5 l/min for an anticipated duration of up to 14 days [1]. It represents the evolution of Impella RD, which required surgical implantation and is no longer available for clinical use.
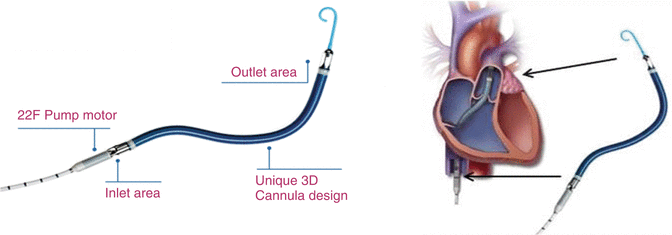
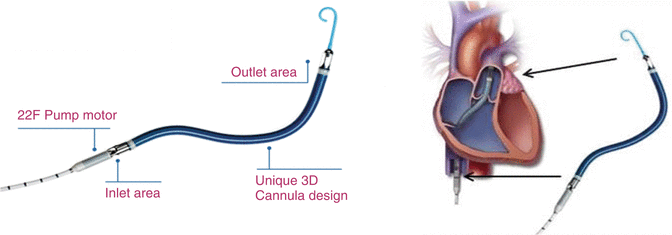
Fig. 12.5
The Impella RP. Note the positioning of the inlet area in the inferior vena cava and of the outlet area into the main PA [Courtesy of Abiomed Inc]
TandemHeart® is a short-term (<14 days) external centrifugal blood pump with a fluid dynamic hydraulic bearing and rotational speed of 2,500–4,500 rpm that provides up to 4 l/min [35]. Its primary use is LV support with the inflow cannula percutaneously inserted transseptal to the left atrium through the femoral vein and the outflow cannula introduced to either femoral artery. As far as RV support is concerned, a long 21 French inflow cannula is inserted percutaneously through the right or left femoral vein and advanced towards the right atrium under fluoroscopic and echocardiographic control. A second long 21 French outflow cannula is inserted into the main PA. Implantation of the outflow cannula into the PA could be performed through the right internal jugular vein or femoral vein [36]. The decision is based on the distance from the femoral venous access site to the fifth intercostal space. In tall patients, the right internal jugular vein is preferred in order to ensure adequate length to reach the main PA (Fig. 12.6). Transesophageal echocardiography is very useful in this setting to confirm proper positioning of both cannulae and avoid RV distention from cannulae malposition [37]. Once the inflow and outflow cannulae are in place, the TandemHeart pump is primed and activated at a flow rate of around 4 l/min. Activated clotting time is maintained >250 s or partial thromboplastin time at 60–80 s.
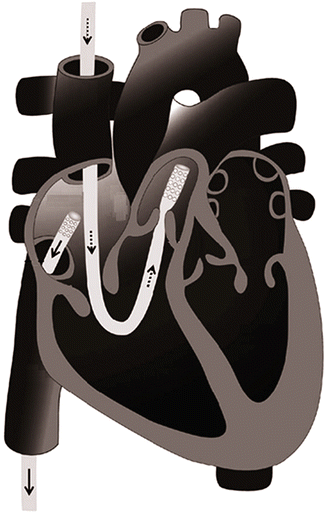
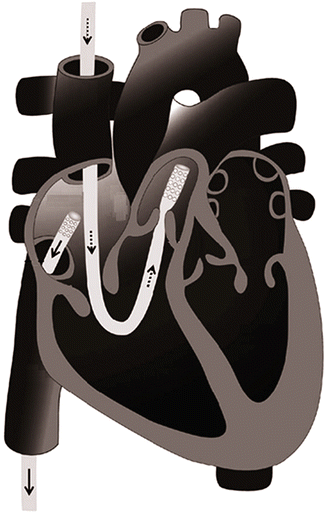
Fig. 12.6
Illustration of TandemHeart inflow and outflow cannula positions within the right heart (Adapted from [37])
Regarding clinical results from the use of percutaneous RVADs, limited data are dispersed in the literature. Impella RP is still under clinical investigation. Margey et al. used the device for temporary MCS in a patient presenting with an inferoposterior acute myocardial infarction, who, despite revascularization, optimized medical therapy, and IABP remained in profound cardiogenic shock [38]. After six days of support, clinical and echocardiographic recovery was evident, and the device was successfully explanted. Cheung et al. described the use of the Impella RP in three patients with refractory ARVF one postcardiotomy, one posttransplant and one post-LVAD, whose devices have been successfully explanted after 7 days of support [1]. Clinical results from the use TandemHeart as RVAD in ARVF are encouraging. Kapur et al. reported in 2011 results of a series of 9 patients with ARVF (6 with inferior-wall myocardial infarction; 2 postcardiotomy and 1 with sepsis) supported successfully by the device [36]. This comprised an overall survival rate of 56 % and augmentation of cardiac output of more than 50 %. Interestingly, the incidence of in-hospital death was higher in RV failure patients in the postcardiotomy setting rather than those with primary PCI after acute inferior-wall myocardial infarction. This could be attributed to the beneficial effect of the reperfusion of the infarcted myocardium additional to mechanical support. The largest retrospective multicenter analysis regarding its use as an RVAD in ARVF included 22 patients managed in 8 tertiary heart centers in the USA [39]. Mean duration of support was 5.4 days. Overall survival rate was 53 %. In general, percutaneous RVADs may be used as bridge to recovery or bridge to surgical RVAD for long-term support.
12.1.5 Surgically Implanted Temporary Blood Pumps
A variety of surgically implanted continuous-flow and pulsatile blood pumps have proven to be effective for temporary MCS [21]. The main advantage of these devices is that they can provide full off-loading (up to 10 l/min) of either or both ventricles (BiVAD). They require surgical central cannulation of atria, ventricles, and great arteries (aorta and main PA) in order to bypass and unload the failing ventricle. Outcome depends on myocardial viability following revascularization, preexisting LV dysfunction, and potential for recovery in stunned or hibernating myocardium. In contrast to percutaneously inserted systems that can be used from 6 h to 14 days, all centrally implanted pumps can be kept in situ for periods ranging from weeks to several months. Temporary pumps can be replaced by long-term implanted LVADs if the native heart does not recover.
The CentriMag ventricular assist system (Thoratec Corporation, Pleasanton, CA, USA) employs a magnetically levitated rotor spinning at 500–5,500 rpm to generate up to 10 l/min of blood flow. The device has no mechanical bearings and no contact between the rotor and pump housing, which eliminates wear on the equipment and results in very low levels of hemolysis or thrombus formation [40]. Patients are heparinized to provide an activated clotting time of >200 s. Immediately after surgery, heparin can safely be withheld for 48–72 h until all mediastinal bleeding resolves. Provided that the flow rate is maintained at >4 l/min, the risks of thrombus formation or thromboembolism are negligible and no antiplatelet agents are required. The pump can be used safely for at least 30 days and, in many cases, for much longer. As implant numbers (>5,000 units to date) and confidence in device reliability increase, support durations of up to 3 months have been described in patients awaiting cardiac transplantation [41]. The system is suitable for both left and right heart support (Fig. 12.7). Ventricular unloading is very effective with a relatively low cost. For these reasons, this system is now widely used throughout Europe and the USA. A recent meta-analysis published by Borisenko et al. included 53 observational studies on patients supported with CentriMag for temporary treatment of refractory cardiogenic shock. LV support was used in 93 patients, RV support in 198 patients, and biventricular support in 179 patients [43]. Thirty-day survival was 66 % in non-cardiotomy cardiogenic shock, 41 % in postcardiotomy cardiogenic shock, 54 % in posttransplant graft rejection or failure, and 61 % in post-LVAD implantation RV failure. In a retrospective review of 29 patients in whom the CentriMag system was used for RV support, Bhama et al. reported a 48 % 30-day mortality [16]. Generally, evidence suggests that immediate introduction of CentriMag support leads to recovery of heart function and weaning from device in a significant proportion of patients [44]. In patients who are stable but not showing an improved cardiac function and in whom multisystem organ failure has been resolved, a decision about heart transplantation or placement of a permanent VAD can be made. By salvaging with CentriMag support, physicians have time to assess the dynamic of the clinical condition and make informed decisions about referring the patient to a heart transplant or permanent VAD implantation.
According to Moazami et al., bridge to recovery can be accomplished in a substantial number of patients with postcardiotomy RV dysfunction with a short period of mechanical support. The RV will recover to an extent that adequate cardiac output can be generated despite significant RV dysfunction. The duration of mechanical therapy was usually less than 1 week during which the RV seems to “adapt” or recovers [45]. Compared to ECLS, centrifugal pumps have the following drawbacks: (i) need for direct surgical cannulation and (ii) circulatory support as compared to circulatory and respiratory support. However, they are associated with much improved unloading of the LV in BiVAD support, and they can be used for longer period compared to ECLS (up to 28 days or even more through recent data) [46].
Need for medium-term support in ARVF (1 month to 1 year) mandates the use of portable pneumatic VADs. The AB5000 (Abiomed Inc.) paracorporeal ventricle is driven by a pneumatic console that allows for patient ambulation and delivers up to 6 l/min of blood flow. The newest portable console offers patient discharge capability. This system can support the left or right heart or both ventricles. Right heart support is performed via the right atrium and PA (Fig. 12.8). Left heart cannulation is achieved through the left atrium or ventricular apex, delivering blood to the ascending aorta. Anticoagulation is recommended with heparin to maintain an activated clotting time of 180–200 s or a partial thromboplastin time of 1.5–2.0 times control values. Other pneumatically driven pumps with similar use include Thoratec paracorporeal VAD (Thoratec PVADTM Thoratec Corporation, Pleasanton, CA, USA) and Excor VAD (Berlin Heart, Berlin, Germany). The latter is also indicated for pediatric patients offering pumps of variable volume. They are mainly used as medium-term LV or biventricular support (implantable LVAD or BiVAD) and represent an ideal solution for bridging patients to recovery, heart transplantation, or implantation of a long-term LVAD for destination therapy (DT). In the setting of acute primary RV failure, paracorporeal pumps have limited applicability compared to CentriMag extracorporeal device due to their higher cost and greater complexity at implantation [16].
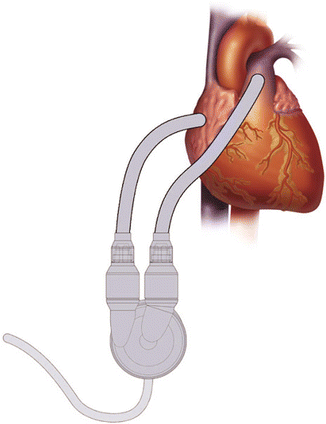
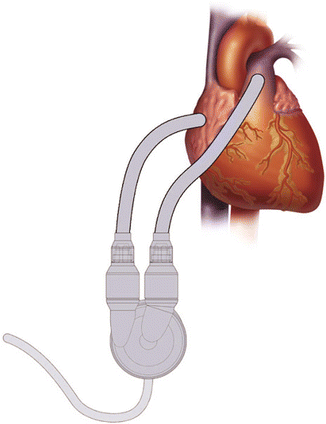
Fig. 12.8
AB5000TM RVAD implantation [Courtesy of Abiomed Inc.]
12.1.6 Decision Making Regarding Temporary RV Mechanical Circulatory Support
The main characteristics of available systems that provide MCS in the setting of ARVF are summarized in Table 12.2. The decision for system selection depends on the etiology of RV failure, expected duration of support, intention to treat, cost, center’s availability, and experience of the team. Taking all these parameters under account, the ECLS represents an easy-to-assemble off-the-shelf solution to conditions such as massive pulmonary embolism, acute myocardial infarction of the RV, and acute fulminant myocarditis, where myocardial recovery after days to weeks of support is anticipated. ECLS offers effective unloading of the RV as well as respiratory support with a relatively low cost. Main use of percutaneously implantable pumps is emergency cases in the catheterization laboratory, where they could provide adequate support of both ventricles.
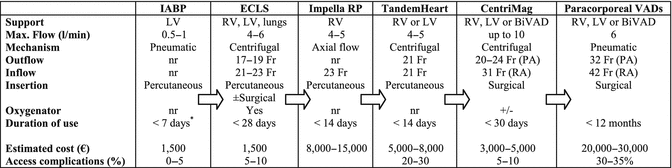
Table 12.2
List of devices used for temporary mechanical circulatory support of the RV
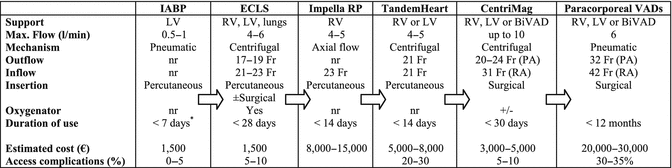
Regarding ARVF postcardiotomy, surgically implantable VADs (extracorporeal or paracorporeal) offer full off-loading of both ventricles and have been used extensively with encouraging clinical results and lower rates of bleeding and infectious complication compared to ECLS. Extracorporeal and paracorporeal RVADs are equally effective in unloading the RV, though the cost of paracorporeal devices is significantly higher compared to ECLS or extracorporeal VADs, which limits their use as a first solution in a limited number of high-volume specialized centers. They are mainly used as a second step to recovery after a temporary VAD has been implanted that supported circulation for 1–4 weeks. Their main advantage is the medium-term duration of support (from 1 month to 1 year) and their portability that offers to the patient the opportunity to recover from critical state and mobilize.
12.2 Right Ventricular Failure Associated with Left Ventricular Assist Device Implantation
Implantation of an LVAD has proved to be a successful treatment option for patients with end-stage heart failure, as either a bridge to transplantation (BTT) or permanent (destination) therapy. However, a significant proportion of patients who undergo implantation with an LVAD develop significant RVF that adversely affects the outcome. The reported incidence of RVF varies between 10 and 30 % for patients after placement of a long-term LVAD, depending on the diagnostic criteria [48, 49]. There is a trend for improvement with evolution of LVAD technology. The latest-generation continuous-flow LVADs are associated with 6–16 % incidence of RVF to their recipients [48, 50, 51]. From these patients, and despite the significant improvements in medical management, use of temporary RV mechanical support is still required in approximately 6–7 % of recipients [48, 52].
Most of the patients with RVF post-LVAD implantation are extremely ill and present with coagulopathy, renal failure, and hepatic congestion. Some of these patients recover successfully on medical therapies supporting the right heart, which include inotropes, phosphodiesterase inhibitors, inhaled nitric oxide (iNO), and prostaglandins; however, 10–15 % require MCS of the RV [10, 53]. When severe RVF occurs, the 1-year mortality of LVAD surgery increases to 44 % compared to 21 % in patients who did not require RVAD support [54, 55]. Moreover, other studies showed higher perioperative mortality, longer length of hospital stay, higher reoperation rates for bleeding, worsened end-organ function, and lower likelihood of successful bridging to transplantation among patients with RVF [53].
Right ventricular failure occurs when transpulmonary flow is unable to provide adequate load to the LVAD. In an attempt to standardize reporting of outcome among different centers, the Interagency Registry for Mechanically Assisted Circulatory Support (INTERMACS) has adopted a definition of RVF following LVAD implantation (Table 12.3) [56]. Three sets of criteria have been identified: (i) evidence of RV dysfunction with cardiac index less than 2.2 l/min/m2 and central venous pressure (CVP) of 18–22 mmHg; (ii) need for postoperative intravenous inotropic support for more than 14 days, iNO for more than 48 h, right-sided circulatory support such as RVAD, or hospital discharge on an inotrope; and (iii) absence of other causes explaining circulatory failure [57]. Imaging criteria of RV dysfunction have not been systematically incorporated in the definition of RVF post-LVAD, in part due to the difficulty in imaging the RV in the early postoperative period. In terms of severity, RVF is graded as severe when requiring RVAD support, moderate when requiring inotropic support or pulmonary vasodilators, and mild when RVF is clinically tolerated by the patient. According to the time period, RVF post-LVAD implantation is classified as intraoperative, early (<14 days) or late (>14 days) [58].
Table 12.3
INTERMACS definition criteria and severity scale of RVF post-LVAD implantation
Diagnostic criteria for RV failure | Symptoms and signs of persistent RV dysfunction, CVP >18 mmHg with a cardiac index <2.0 l/min/m2 |
In the absence of elevated left atrial/pulmonary capillary wedge pressure >18 mmHg, tamponade, ventricular arrhythmias, or pneumothorax | |
Requiring RVAD implantation or requiring iNO or inotropic therapy for duration of more than 1 week at any time after LVAD implantation | |
Severity scale | |
Severe | Need for RVAD |
Moderate | Need for inotrope or intravenous or inhaled pulmonary vasodilators |
Mild | Meets 2 of the 4 clinical criteria |
CVP >18 mmHg or mean RA pressure >18 mmHg Cardiac index <2.3 l/min/m2 | |
Ascites or evidence of peripheral edema | |
Evidence of elevated CVP by echocardiography or physical examination |
Physiological changes affecting the RV post-LVAD implantation are schematically presented in Fig. 12.9. LVAD function has an unpredictable effect on RV function. Successful unloading of the LV requires the native RV to increase its output to match LVAD flow. Augmentation of cardiac output and systemic blood flow by the LVAD increase venous return to the RV. To cope with the increased preload, RV diastolic compliance improves through decreased RV afterload and leftward shift of the interventricular septum as the LVAD reduces LV pressure (Fig. 12.10). RV efficiency is maintained as the unloaded RV does not need to contract as vigorously to eject the increased RV preload [59]. Clinically, this shows as a decrease in pulmonary capillary wedge pressure (PCWP), pulmonary artery pressure (PAP), and peak RV systolic pressure.
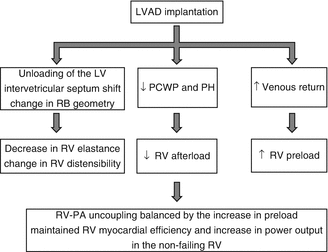
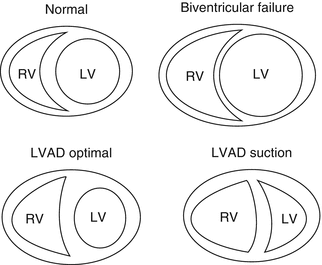
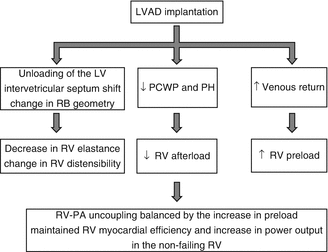
Fig. 12.9
Physiology of RV function after LVAD implantation
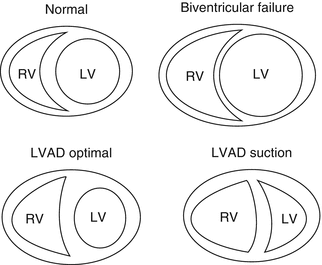
Fig. 12.10
Changes in RV shape after LVAD implantation
The LVAD is both beneficial and detrimental to the RV. Depending on the preexisting condition of the RV, the LVAD may have a beneficial effect by reducing afterload or a detrimental effect by increasing preload to an RV already compromised by cardiomyopathy, ischemia, arrhythmias, or PAH [55]. The anatomical, functional, and physiological parameters imposed upon the RV by an LVAD create a set of conditions whereby the RV operates in a disadvantaged mechanical zone. The ability of the RV to supply the left heart and thus the LVAD with adequate volume is related to intrinsic RV contractility, PVR, and volume status [60]. The LVAD unloads the LV and results in a decrease in the reversible component of PAH, thereby increasing RV preload and stroke volume [61]. On the other hand, as the LVAD unloads the LV, the interventricular septum is shifted left (ventricular interdependence). This results in a more spherical shape of the RV, which alters the RV compliance thereby decreasing force and rate of contraction. The RV-free wall must work harder and cannot compensate for the loss of septal function causing fatigue, which further exhausts the RV. Moreover, changes in RV and septal geometry increase tricuspid annular dilatation and, thus, lead to either onset or worsening of preexisting tricuspid regurgitation [62]. Tricuspid valve annulus may be distorted by high LVAD flows. Furthermore, increased PVR post-CPB related to systemic inflammatory response syndrome as well as blood product transfusion (mediated by thromboxane A2, cytokines IL-1β, IL-6, IL-10, and TNF-α) contributes to RV dysfunction [63–65].
12.2.1 Predictors of RVF Post-LVAD Implantation
Many authors have attempted to identify preoperative risk factors and, hence, develop risk scores to predict LVAD candidates who may develop postoperative RVF. This high-risk group of patients may benefit from preoperative optimization of right heart function or from planned rather than unexpected BiVAD support. However, because of its multifactorial nature, postoperative RV dysfunction remains difficult to predict in individual potential LVAD candidates [59]. A variety of clinical, laboratory, echocardiographic, and hemodynamic parameters have been associated with RVF following LVAD implantation in several studies [66]. These parameters are summarized in Table 12.4. It should be noted that predictors of RV dysfunction pre- and post-LVAD implantation are neither fully sensitive nor specific, and, hence, they have not been incorporated into clinical practice guidelines [67]. For this reason, widespread clinical implementation is low [68].
Table 12.4
Preoperative predictors of RVF following LVAD implantation
Clinical predictors |
Nonischemic etiology of cardiomyopathy |
Female gender |
Younger or older age |
Preoperative IABP |
Mechanical ventilation pre-LVAD |
Previous cardiac surgery |
Low BSA |
Emergent operation |
Previous mechanical circulatory support |
Echocardiographic predictors |
Tricuspid annular diameter >43 mm |
Severe tricuspid regurgitation |
TAPSE <7.7 mm |
LVEDD <63 mm |
RV/LV diameter ratio >0.72 |
RV short-/long-axis ratio ≥0.6 |
RV peak strain <−9.6 % |
Hemodynamic predictors |
Elevated right atrial pressure |
CVP/PCWP ratio >0.63 |
RVSWI <300–600 |
Low mean pulmonary artery pressure |
Low cardiac index |
Laboratory predictors |
Elevated bilirubin levels |
Elevated serum creatinine levels |
Blood urea nitrogen >39 mg/dl |
INR >1.1 |
Elevated AST levels |
Albumin ≤3.3 g/dl |
Elevated NT-proBNP |
Elevated WBC |
Low platelet count |
Moreover, the “vulnerable” RV and the “frail” patient may ultimately lead to RVF post-LVAD implantation as illustrated in Fig. 12.11. Analyzing Table 12.4, in order to identify the “vulnerable” RV, echocardiographic parameters have gained paramount importance [57], as analyzed in Echocardiography chapter. Preoperative echocardiographic evaluation of RV function is a critical component of risk stratification for postoperative RVF following LVAD implantation. Qualitative assessment of global RV ejection fraction (RVEF), despite having significant variability, has been recognized as an independent predictor of the need for biventricular support [69]. Given the limitations of qualitative global assessment of RVEF, a number of quantitative echocardiographic variables have been investigated to assess RV function, such as tricuspid annular motion (TAPSE). Preoperative TAPSE of <7.5 mm predicted post-LVAD RV failure with nearly 90 % specificity and 50 % sensitivity [70]. Moreover, a tricuspid annulus diameter >43 mm, as determined by transesophageal echocardiography (TEE) in the mid-esophageal 4-chamber view, was associated with an increased risk of RVF and 1-year mortality post-LVAD [71]. Kukucka et al. reported increased preoperative ratio of RV to LV size in patients with LVAD who developed postoperative RVF; the same pattern applied to patients with BiVAD support: RV/LV ratio of >0.72 or short/long RV ≥0.6 predicted 30-day survival with a sensitivity more than 60 % and a specificity more than 70 % [71, 72]. Moreover, there is a strong correlation between severity of tricuspid regurgitation and incidence of postoperative RVF (odds ratio: 4.7) [72]. Furthermore, a cutoff for RV peak strain of <−9.6 % was found to predict the incidence of postoperative RVF (sensitivity around 70 % and specificity more than 75 %) [73].
Regarding hemodynamic parameters, increased preoperative or intraoperative CVP (>16 mmHg) and decreased RV stroke work index (<300 mmHg ml−1 m−2) may identify the vulnerable RV [48, 74]. Moreover, PAP is an indicator of RV contractility and may predict post-LVAD RVF. Patients with low preoperative PAP are more likely to develop RVF post-LVAD implantation. This reflects inability of RV to overcome the elevated PVR. Low preoperative RV performance (low PAP or RV stroke work index) may predict the postoperative need for RVAD or BiVAD support.
Preoperative end-organ damage, as manifested with clinical and laboratory parameters, may contribute to the identification of the “frail” patient. Patients with evidence of multiorgan dysfunction preoperatively (on ventilatory support, on IABP support, or with liver dysfunction, renal failure, and coagulation impairment) are at higher risk of developing RVF [48, 73–75]. In general, female gender has been found to be an independent risk factor for developing RVF post-LVAD [76]. Interestingly, Drakos et al. found that intention to treat DT rather than bridge to transplantation is a risk factor for developing RVF. This may be due to the fact that patients selected for DT are usually more ill, they have more comorbidities, and they are selected for treatment later in the disease process [77]. Moreover, nonspecific biochemical markers of heart failure, such as N-terminal pro-brain natriuretic peptide (NT pro-BNP) and neopterin, as well as the inflammatory markers procalcitonin and big endothelin-1 were found to be more elevated preoperatively in patients developing RVF post-LVAD [78].
In summary, as illustrated in Table 12.5, there are several models derived from retrospective analyses designed to predict the risk of RVF post-LVAD implantation. Even though these scoring systems represent attractive predictive models, they need to be prospectively validated in the current era of clinical practice with new-generation devices. In any case, they provide useful tools for preoperative evaluation of patients allocated to mechanical support [66]. The early models developed to predict RV failure were based on the analyses of patients with pulsatile first-generation LVADs. Lietz et al. initiated the Destination Therapy Risk Score (DTRS), derived from an analysis of 280 patients implanted with the HeartMate XVE LVAD used for DT [79]. Fitzpatrick et al. developed another model based on a retrospective analysis of 266 patients implanted with a variety of pulsatile LVADs [69]. These models showed remarkable sensitivity and specificity in predicting postoperative RVF; however, their role in predicting need for RV support in patients implanted with continuous-flow devices remains unknown.
Table 12.5
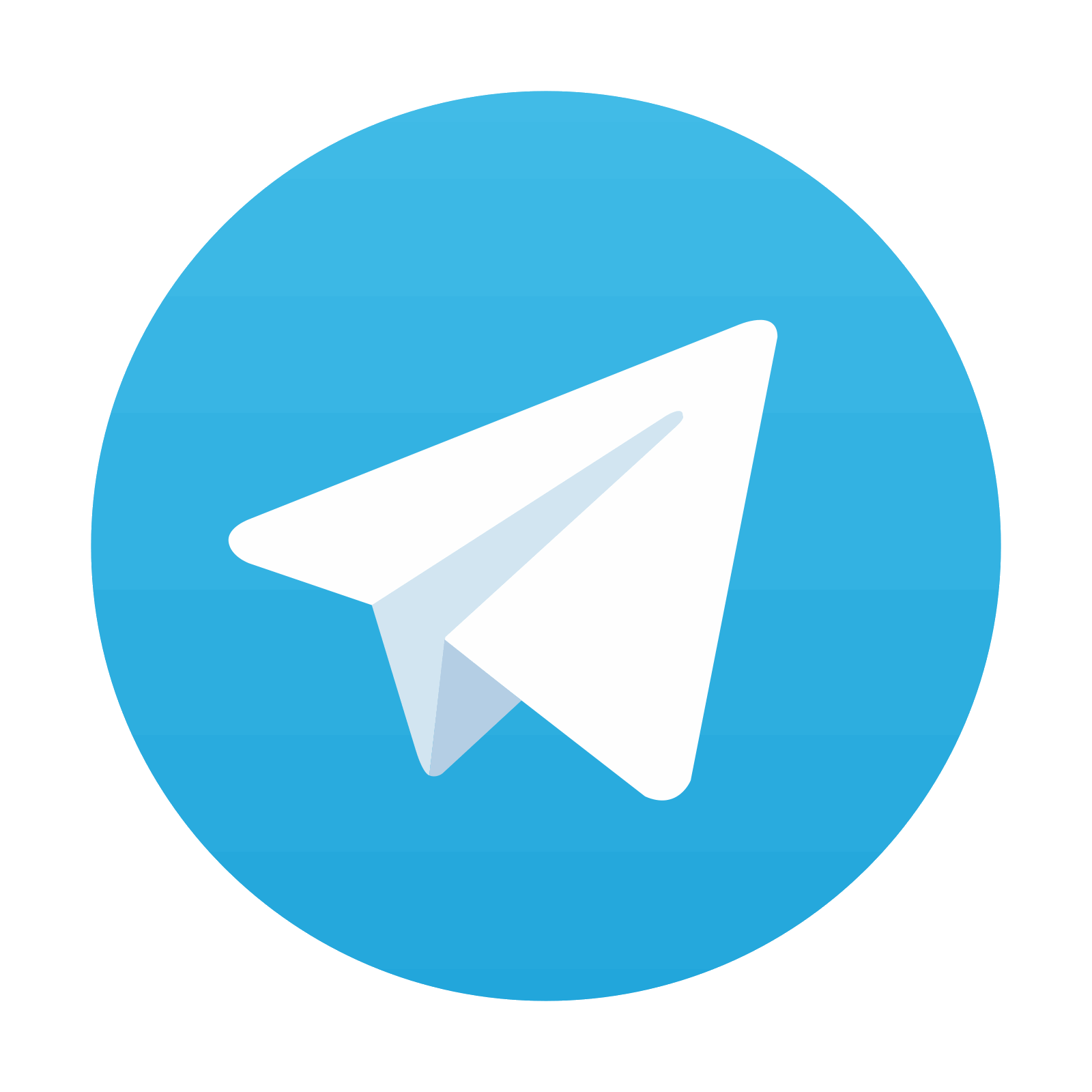
Risk prediction models for RVF post-LVAD implantation
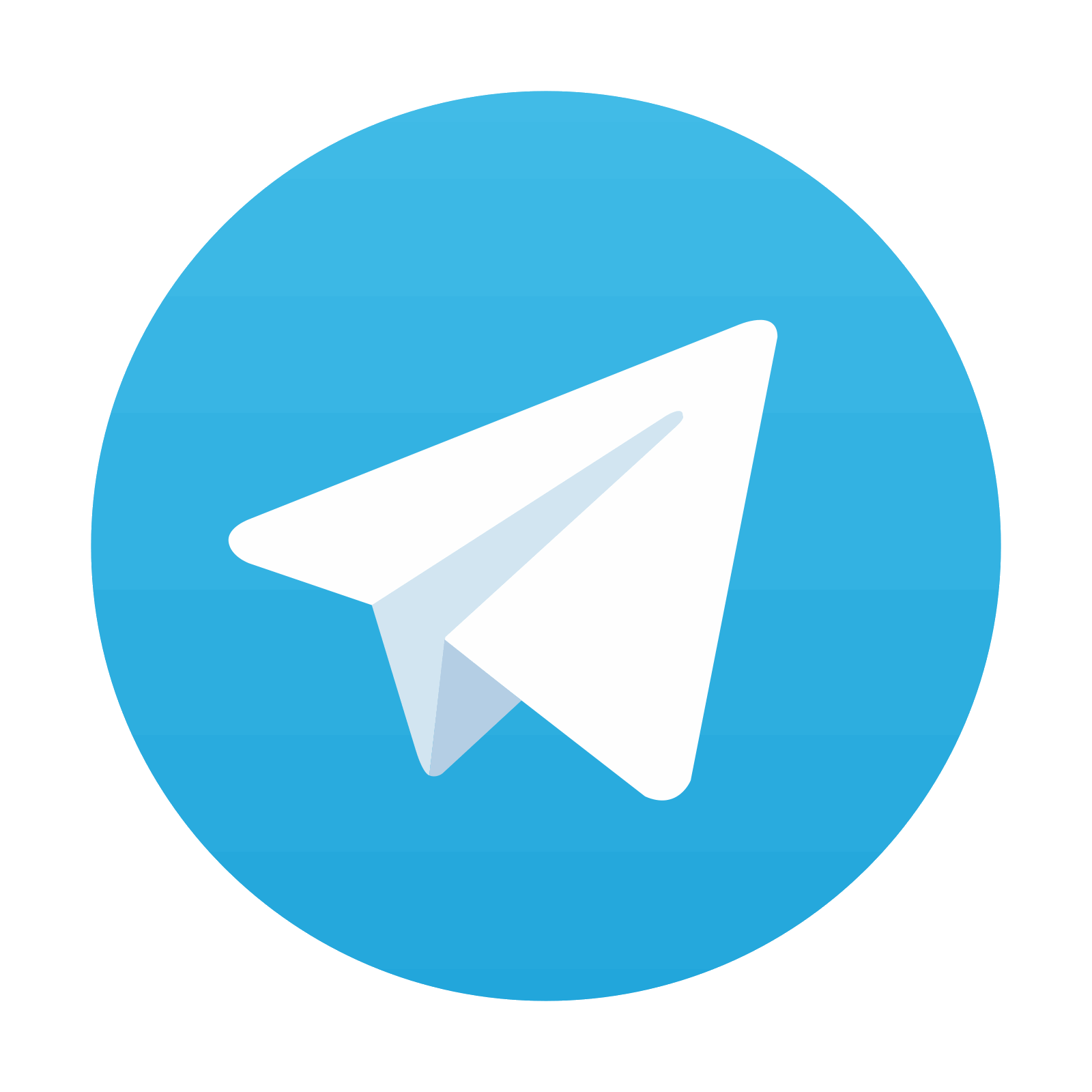
Stay updated, free articles. Join our Telegram channel
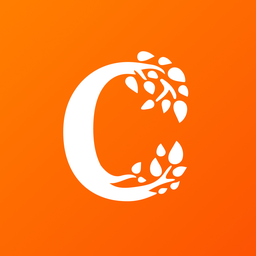
Full access? Get Clinical Tree
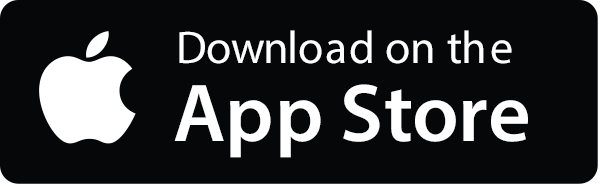
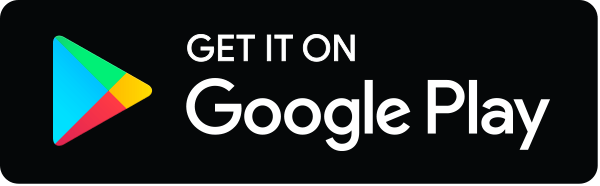
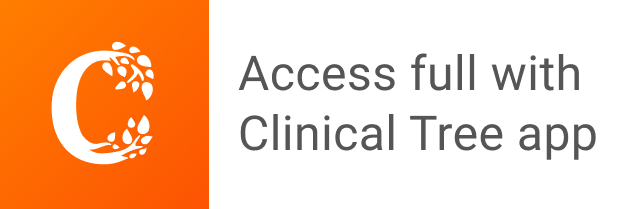