Magnetic resonance imaging (MRI) is an imaging modality for venous diseases that is an alternative to more conventional techniques such as ultrasonography, impedance plethysmography, computed tomography (CT), and invasive selective contrast venography. The advantages of MRI include its noninvasive nature, lack of ionizing radiation and exposure to iodine-based contrast, high spatial resolution, ability to acquire unlimited imaging planes, and its unique ability to perform multicontrast tissue characterization. Despite these advantages, however, routine magnetic resonance venography (MRV) is not widely performed because it generally requires a radiologic team that is familiar with this specialized technique. Also, MRV lacks portability and sometimes incur a higher cost compared with other conventional techniques.
MRI is based on the exploitation of the magnetization properties inherent in hydrogen protons of tissues to acquire images. In the presence of a strong external magnetic field, more commonly a 1.5-Tesla or increasingly 3.0-Tesla magnet, the hydrogen protons in the body precess along the direction of the magnetic field. Brief external radiofrequency (RF) pulses with a magnitude dependent on a proton’s gyromagnetic ratio (an intrinsic property of the proton) and the field strength are applied, causing a disruption of the alignment, magnetization of the hydrogen proton, and a net absorption of energy. This pulse is rapidly turned off, and the protons return to their original alignment with the magnetic field. This shift from a higher energy to a lower energy state creates RF waves that are detected by receiver coils adjacent to the body and are converted into electrical signals that form the basis of an MRI image. Spatial localization of the signal is accomplished through gradient coils that produce subtle regional differences in resonant frequency in multiple directions.
MRV can be divided into two major groups: noncontrast MRV and contrast-enhanced MRV based on the use of gadolinium-based contrast agents. Noncontrast MRV, in turn, is performed most commonly using time-of-flight (TOF) or phase contrast (PC) techniques.1
This is a type of MRI that relies on gradient echo or “bright blood” imaging. When subjected to a long series of RF pulses with a short echo time, flowing blood shows signal enhancement because motion allows these moving protons to experience only a restricted number of RF excitations before moving out of the imaged volume, and the continuous refreshment or inflow of spins into the imaging volume ensures a maximal alignment of protons with the external magnetic field before the RF pulses.2 In contrast, stationary tissues have partially relaxed signals that lead to lower signal intensity. Even without the use of contrast, the TOF images show hyperintense signals in the vessels, and the technique is robust as long as the protons in the blood experience only a few RF pulses before being refreshed. To differentiate venous from arterial signal, a presaturation band that nulls inflowing arterial blood proton spins is placed upstream in the direction of arterial flow, effectively reducing the signal intensity of blood flow within the arteries of the volume of tissue being imaged.1,3
The signal intensity of moving blood is greatest when blood flow is perpendicular to the imaging plane and least when it is parallel to the plane, the latter caused by signal loss from spin saturation. Therefore, careful prescription of the imaging plane is essential to obtain optimal diagnostic images. The flip angle of the RF pulse is important: a reduced flip angle minimizes in-plane flow saturation, and a flip angle of 20° to 25° is optimal for in-plane flow, but a higher flip angle of 45° is ideal for through plane flow.1,3 TOF can be performed in two- dimensional (plane) or three-dimensional (volume) acquisitions. Whereas two-dimensional TOF is ideal for venous imaging because of detection of slow flow, sensitivity to T1 effects, shorter (but multiple) breath holds, and large area of coverage, three-dimensional TOF has advantages in terms of higher spatial resolution and reduced saturation of in-plane flow.1
A special type of gradient echo sequence, PC, involves introduction of extra bipolar pulses that allows proton spins to acquire a phase angle that is proportional to the velocity of the protons. The velocity of moving blood can therefore be measured from the phase angles.2 The phase effect can be used to study flow patterns and the strength of the velocity encoding bipolar pulse (VENC) determines the maximum velocity that can be measured by this technique. The recommended VENC is slightly higher than the fastest velocity in the vessel of interest; for venous imaging, it is around 20 cm/s.1 PC imaging is an MR angiographic technique that involves taking simultaneous phase compensated images and phase encoded images with bipolar pulses along the x, y, and z directions. By subtracting the signals induced by spins in the encoded series from the compensated series, one is left with only signals from the vessels. Although more time consuming than ordinary MR imaging, it is an efficient way of suppressing the signal of stationary tissues to image the blood vessels. Similar to TOF imaging, acquisition can be either two or three dimensional. Advantages of PC MRV over TOF include quantification of flow velocity and flow patterns, enhanced background suppression, sensitivity to various vascular velocities, reduced intravoxel dephasing and simultaneous acquisition of magnitude, and phase images that allows structural and functional correlation. An example of a PC image acquisition can be seen in Figure 9-1.
FIGURE 9-1.
Phase contrast magnetic resonance imaging (MRI). A. Velocity-encoded image of a phase contrast MRI depicts flow in the great vessels and cardiac chambers. White and black areas represent through-plane flow (toward or away from the imaging plane) with signal intensity related to flow velocity, and mottled gray areas represent relatively fixed structures. Postprocessing software can be used to calculate flow velocity and pressure gradients using phase information. B. Simultaneous magnitude image corresponding to the cardiac anatomic structures. Ao, aorta; RV, right ventricle.

Unlike TOF and PC techniques that rely on blood flow or refreshment of fresh signals from moving blood, flow-independent techniques have been described that exploit the T1, T2, and chemical shift differences to suppress background tissue and enhance venous blood.4,5,6 The T2 of blood decreases with decreasing oxyhemoglobin and therefore lower in venous than arterial blood. By exploiting this as well as tissue differences in contrast weightings between venous blood, muscle, and lipids, excellent depiction of venous anatomy was achieved in six volunteers.4 A three-dimensional fresh blood imaging method that reduces the echo interval of the fast advance spin-echo sequence was used to delineate arteries and veins in 14 patients with venous disease.6 The sensitivity of the technique was 100% in all anatomical levels in which deep venous thrombosis (DVT) was diagnosed by contrast venography, and the technique had superior delineation of collateral veins compared with two-dimensional TOF imaging. This technique, however, remains to be extensively used or further validated clinically.
Gadolinium is a paramagnetic contrast agent that shortens tissue T1 relaxation time, providing high signal for vascular contrast enhancement.7 Contrast MRV relies on recirculation of contrast and can be performed using either indirect or direct approach.1 With the indirect approach, nondiluted contrast is injected in a nontargeted vein or extremity and imaging is acquired during the first pass in the early equilibrium phase to avoid redistribution. The direct approach, on the other hand, involves injection of dilute contrast in a vein proximal to the area of interest followed by imaging of the draining venous system, a process similar to conventional iodine-based venography. A dilution of 1:20 with saline is typically administered.1,8 The direct approach is limited in its applicability to those veins that drain from the site of injection, although it provides superior contrast-to-noise ratio. Contrast-enhanced MRV does not provide good-quality images of the calf veins.5 but images are improved with digital subtraction methods.9 Three dimensional gadolinium-enhanced MRV was performed in 37 patients with varying central venous pathologies (thromboses, stenoses, and compression) and compared with conventional imaging (Doppler sonography, CT, or digital subtraction venography). It was found to be 100% sensitive, specific, and accurate in the diagnosis.10 An example of three-dimensional pulmonary venous angiography with slice reconstruction showing pulmonary vein stenosis after atrial fibrillation ablation is shown in Figure 9-2. There are promising newer contrast agents such as gadofosveset that bind strongly but reversibly to albumin and remain in the vascular compartment longer, enhancing imaging of veins that take longer to fill because of slow flow.5,11,12
FIGURE 9-2.
Pulmonary vein stenosis after atrial fibrillation ablation. A. Pulmonary vein magnetic resonance image with maximum intensity projection image in the coronal view showing the left atrial structure, the pulmonary venous system, and the pulmonary arterial structure. B. Multiplanar reconstruction demonstrating the left atrium (LA), the right pulmonary vein, and the left upper pulmonary vein. The ostium of the left upper pulmonary vein has ostial narrowing and stenosis after the atrial fibrillation ablation procedure (arrow).


Combined arterial and venous magnetic resonance angiography (MRA) has been proposed for comprehensive examination in patients with arterial thrombi that would include investigating sources for paradoxical embolism in the deep venous system (Figure 9-3).13 Three-dimensional contrast-enhanced whole-body angiography using five overlapping three-dimensional coronal datasets acquired in immediate succession of three-dimensional fast low-angle shot gradient recalled echo-pulse sequences was found to be feasible in 10 subjects with whole-body venography performed immediately after the acquisition of the arterial datasets. In a study of 20 patients with pulmonary embolism (PE) and DVT, a combined approach of visualizing the pulmonary arteries and deep lower extremity venous system using contrast-enhanced MR angiography yielded 90% concordance for PE versus CT or perfusion lung scan and 75% concordance with duplex sonography or contrast venography for DVT.14 This study supports the potential of a “one-stop shop” for venous thromboembolism.
FIGURE 9-3.
Combined whole-body arterial, venous, and cardiac magnetic resonance image in thromboembolic disease. The images are from a 26-year-old woman with painful leg swelling and acute dyspnea. A. Lower leg magnetic resonance angiography (MRA) reveals occlusion of the distal runoff arteries on both sides (arrows) with mild venous overlay. B. Pulmonary MRA reveals pulmonary embolism in the left upper and lower lung lobe artery (arrows). C and D. Magnetic resonance venography (late phase of contrast agent dilution) demonstrates renal and spleen (arrows) infarction. E. High spatial resolution venography demonstrates thrombi in the popliteal vein and artery of the left leg (arrows). F. Coronal reformatted MRA of the lower legs shows the extent of thromboembolic formation (arrows). Echocardiography revealed the presence of a patent foramen ovale.
(Reprinted with permission from Vogt et al.13)

Three-dimensional acquisition provides superior spatial resolution, and use of gadolinium contrast allows enhanced contrast-to-noise ratio in the vessels of interest. Postprocessing techniques allow the visual display of complex vascular anatomy. The most useful algorithms are multiplanar reconstruction (MPR), maximum intensity projection (MIP), and volume rendering.1 MPR involves extraction of a two-dimensional projection from three-dimensional volume information, and the algorithm allows cross-sectional interrogation of the three-dimensional data in unlimited planes, allowing for visualization of complex vascular relationships. MIP involves passing a projection ray through the volume data so that the data are projected on a two-dimensional plane. A projective image is calculated by penetrating the data volume with a set of parallel projection rays and selecting only the data that represent the maximum intensity for each of the rays.1 The process is repeated at different angles, and the images formed are combined to produce a three-dimensional representation of the vascular structures. Direct volume rendering involves a two-step process of classification and rendering. Classification involves assigning brightness and color to each voxel of a particular tissue type and assigning partial transparency to the tissue of signal-intensity classes. Rendering involves image projection to form a three-dimensional image. An example of MIP and MPR of the pulmonary venous system is shown in Figure 9-2. An example of volume rendering is seen in Figure 9-4.
FIGURE 9-4.
Partial anomalous pulmonary venous drainage. A. Steady-state free precession gradient-echo oblique coronal view demonstrating the anomalous entry of the right upper pulmonary vein (arrowhead) to the superior vena cava. B. Oblique sagittal view of the atria demonstrates connection between the right atrium (RA) and left atrium corresponding to a sinus venosus atrial septal defect. C. Volume-rendered three-dimensional image shows the anomalous entry of the pulmonary vein into the superior vena cava.

It is estimated that venous thromboembolism account for nearly 200,000 hospitalizations annually in the United States, with nearly an equal number of cases of first-time venous thromboembolism diagnosed yearly.15,16 In a large community-based study, the age-standardized incidence of first-time DVTs has been estimated at 1.92 per 1000.16 PE is the major complication of DVT, which is frequently under diagnosed. In one large review of death certificates, 1.3% of all deceased had a diagnosis of PE.17 Upper extremity venous thromboses account for 10% of all DVTs and are increasing in incidence secondary to use of upper extremity venous access catheters for either management of malignancies or dialysis.18
DVTs originate most commonly in the deep veins of the calf, particularly the deep muscular veins draining soleus and gastrocnemius muscle. About 20% of these will spread proximally to popliteal vein or above.18 Virchow’s triad (stasis, hypercoagulability, and trauma to endothelial lining) predispose to the development of DVT. Hence, multiple risk factors for DVT have been described, including age, trauma, obesity, history of DVT, immobilization, recent surgery, malignancy, use of oral contraceptive pills, pregnancy, hypercoagulable state, and stroke.
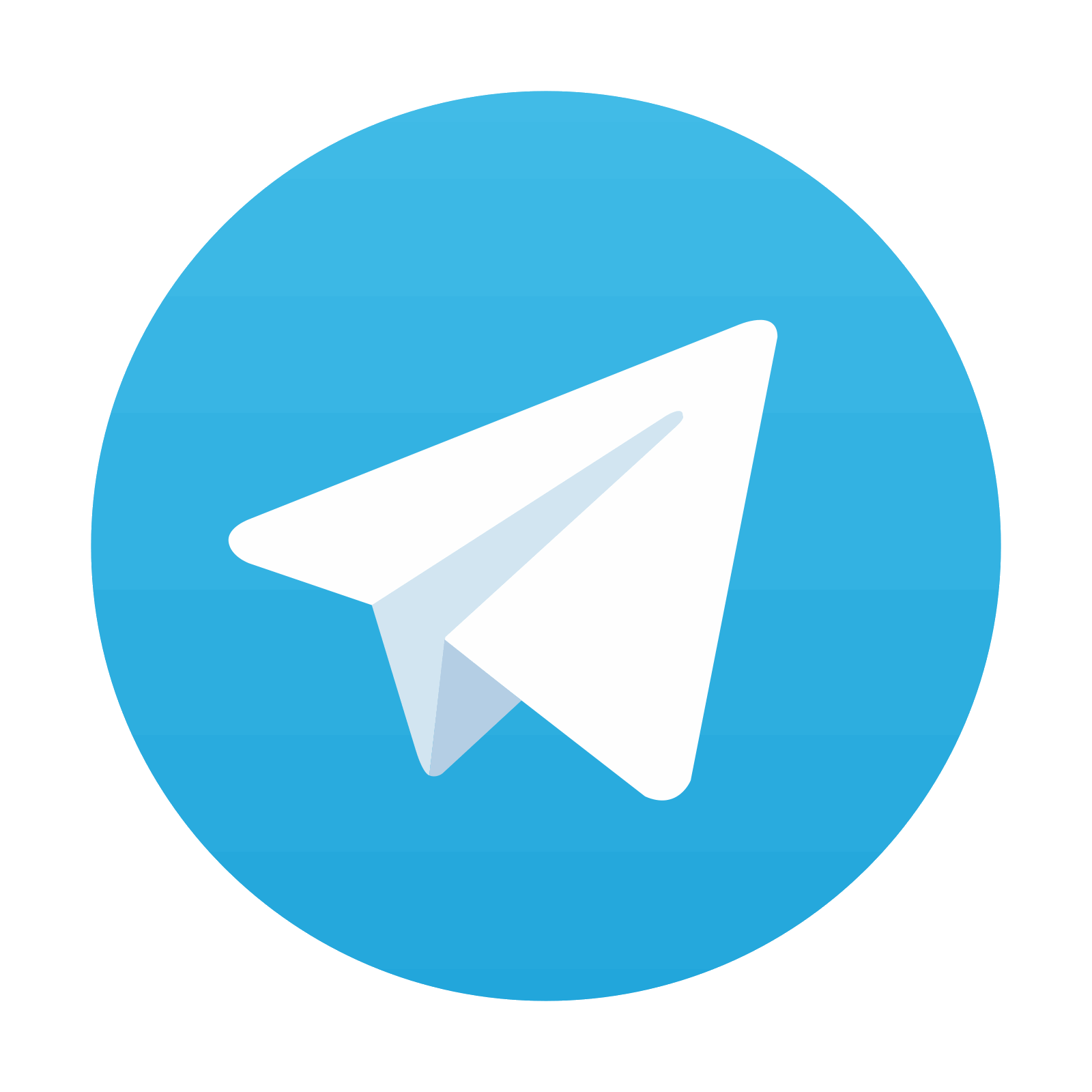
Stay updated, free articles. Join our Telegram channel
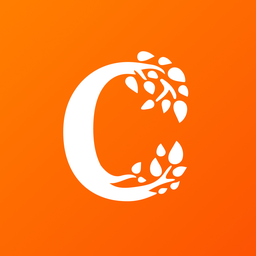
Full access? Get Clinical Tree
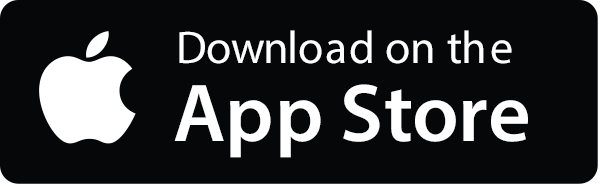
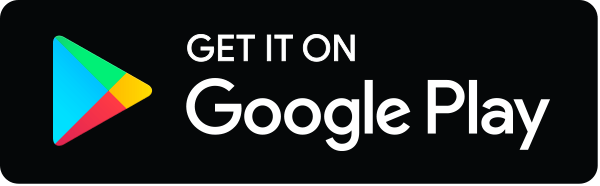