Magnetic Resonance Imaging of Function and Flow in Postoperative Congenital Heart Disease
Arno A.W. Roest
Lucia J.M. Kroft
Albert de Roos
Congenital heart disease (CHD) survival rates have increased dramatically because of improvements in diagnostic tools, surgical techniques, and postoperative care for patients with CHD during the last 40 years. Traditionally, these patients are monitored over time by clinical assessment, electrocardiography, echocardiography, and x-ray angiography. Transthoracic echocardiography is the most commonly used technique. However, after surgical intervention the imaging quality is often restricted because scar, bone, or lung tissue may interfere with the acoustic window, while chest deformations may be present as well. X-ray angiography has advantages for vascular imaging quality, but overprojection of structures, radiation burden, and invasiveness are important drawbacks. These limitations do not occur with magnetic resonance imaging (MRI). With this imaging technique, both superior depiction of cardiac anatomy and reproducible measurement of cardiac function can be achieved in one session. Over the last 15 years MRI has been increasingly accepted as an important imaging tool for assessment of postoperative cardiovascular anatomy and function in patients with corrected or palliated CHD.
The focus of this chapter is to discuss the current role of MRI in the evaluation of patients with CHD after correction or palliation. This is of paramount importance as many patients have residual defects after intervention or will develop cardiovascular dysfunction during follow-up. Technical options that are available with MRI are described, and the practical applications of these options will be discussed for the four most common referrals: Tetralogy of Fallot (TOF), transposition of the great arteries (TGA), aortic coarctation, and the Fontan circulation.
TECHNICAL OPTIONS
A wide array of MRI techniques is available for detailed determination of cardiac morphology and quantification of cardiac function. The technical aspects have been discussed in more detail in other chapters of this book.
BLACK BLOOD IMAGING
Black blood imaging provides clear depiction of the cardiac anatomy. The electrocardiographically triggered multislice spinecho acquisition is performed during breath-holds. The black blood appearance is caused by a double-inversion pulse with an appropriate delay, creating a signal void for flowing blood (black) with high tissue-to-blood contrast. It provides twodimensional (2D) images in three different directions (sagittal, transverse, and coronal) and in any other direction tailored to the morphology (1). Thin-slice (3 to 5 mm) imaging is preferred to avoid partial volume effects. For example, the assessment of focal stenosis as in coarctation of the aorta is best accomplished with 3-mm slice thickness in double-oblique planes along the course of the vessel of interest.
BALANCED GRADIENT-ECHO IMAGING AND SENSE TECHNOLOGY
Gradient-echo balanced fast-field echo techniques can be used to assess the function of both ventricles in a highly
reproducible way (2,3 and 4). A stack of consecutive slices can be applied in the transverse plane or in short-axis orientation, covering both ventricles. Endocardial and epicardial borders can then be traced by using a dedicated software package. Parameters like end-diastolic volume (EDV) and end-systolic volume (ESV), stroke volume (SV), cardiac output, ejection fraction (EF), and wall mass (the latter to assess the degree of myocardial hypertrophy) can be obtained. Wall motion abnormalities can be easily depicted when these images are displayed in movie-loop. Gradient-echo multislice multiphase MRI also allows identification of recurrent or residual septal defects by displaying a signal void at the site of turbulent and/or high-velocity flow through a defect. The signal void is caused by dephasing of the protons within a jet, resulting in a local loss of signal intensity observed (Fig. 30.1). Such flow voids can also indicate valvular regurgitation or stenosis.
reproducible way (2,3 and 4). A stack of consecutive slices can be applied in the transverse plane or in short-axis orientation, covering both ventricles. Endocardial and epicardial borders can then be traced by using a dedicated software package. Parameters like end-diastolic volume (EDV) and end-systolic volume (ESV), stroke volume (SV), cardiac output, ejection fraction (EF), and wall mass (the latter to assess the degree of myocardial hypertrophy) can be obtained. Wall motion abnormalities can be easily depicted when these images are displayed in movie-loop. Gradient-echo multislice multiphase MRI also allows identification of recurrent or residual septal defects by displaying a signal void at the site of turbulent and/or high-velocity flow through a defect. The signal void is caused by dephasing of the protons within a jet, resulting in a local loss of signal intensity observed (Fig. 30.1). Such flow voids can also indicate valvular regurgitation or stenosis.
So-called SENSE (SENSitivity Encoding) technology allows considerable scan-time reduction in most MRI techniques, including black blood spin-echo and balanced fast-field gradientecho imaging. Simultaneously operated coils are used to improve the signal-to-noise ratio. New techniques like the recently introduced k-t broad-use linear acquisition speed-up technique (k-t BLAST) will also provide increased spatial and temporal resolution, in which a complete stack of short-axis images can be obtained in a single breath-hold (5). These faster imaging sequences will especially be of benefit for the pediatric population, because small children often have difficulties with holding their breath.
FLOW MAPPING
Assessment of valvular and vascular function with phasecontrast MRI is another essential tool, allowing accurate measurement of regurgitation and/or stenosis at any level in the heart. With phase-contrast MRI the flow velocity of blood can be measured, based on velocity-induced phase shifts of moving protons in the presence of a magnetic field gradient. Across the area of interest, flow volumes of both the forward flow and any regurgitation can be assessed, whereas peak velocities can be used to estimate pressure gradients over stenotic segments, using the modified Bernoulli equation. The advent of three-dimensional (3D) flow mapping with retrospective valve tracking allows more detailed evaluation of valvular function. Valve tracking is especially important to study flow over the tricuspid en mitral valve, as they move up to 24 mm during ventricular contraction (6). Based on this technique flow mapping can also provide information on the diastolic filling pattern of the ventricle and allows the construction of a ventricular time-volume curve (Fig. 30.2) (7). In patients with corrected TOF and pulmonary regurgitation (PR), right ventricular (RV) inflow curves have been used to demonstrate impaired relaxation and restriction to filling as markers of abnormal RV diastolic function (7). Shunt quantification in the case of a left-to-right shunt is possible by comparing the aortic and pulmonary net forward flow (8,9 and 10). It can also be assessed by comparison of the RV and left ventricular (LV) SVs calculated from gradient-echo imaging, with the exception of the presence of a ventricular septal defect (VSD), because RV and LV SVs appear to be the same then. Furthermore, shunt quantification is complicated when multiple shunts are present or with coexisting valvular regurgitation. More recently phase-contrast MRI was used to assess myocardial velocity, providing information on regional systolic and diastolic peak velocities and differences in timing of these events within the left and right ventricle, which is of importance if resynchronization therapy is considered (Fig. 30.3) (11,12).
![]() Figure 30.2. The use of a 3D-velocity-encoded MR imaging sequence with retrospective valve tracking allows assessment of all four cardiac valves during one acquisition. Figure shows post-processing of flow through the tricuspid valve (TV) of a healthy subject. A,B: Cine images show retrospective tracking of the TV performed by positioning the plane of interest perpendicular to the flow through the TV during every cardiac phase in four-chamber (A) and two-chamber (B) views. C–E: Velocity encoded MR images from three orthogonal directions, reconstructed from the position of the valve determined with retrospective valve tracking based on views in (A) and (B). AP, anterior-posterior velocity encoded; FH, feet-to-head velocity-encoded; RL, right-left velocity encoded. F: Through-plane velocity-encoded MR images are obtained by reformatting of center valvular plane in each cardiac phase. Inner border of tricuspid annulus is traced for flow analysis. Region within free wall of right ventricle is traced for background correction. G: Tricuspid flow velocity (in time) is presented in a curve, from which flow volume can be calculated. (Reprinted from van der Hulst AE, Westenberg JJ, Kroft LJ, et al. Tetralogy of fallot: 3D velocity-encoded MR imaging for evaluation of right ventricular valve flow and diastolic function in patients after correction. Radiology. 2010;256(3):724—734, with permission.) |
CONTRAST-ENHANCED MAGNETIC RESONANCE IMAGING
Magnetic Resonance Angiography
Gadolinium-enhanced magnetic resonance angiography (MRA) is well suited to detect morphologic abnormalities of the great vessels, like pulmonary branch stenoses in TOF and coarctation of the aorta (Fig. 30.4). The gadolinium shortens the T1-relaxation time of blood, resulting in a high signal intensity of the blood pool. This allows rapid 3D acquisition of the entire thoracic aorta within one breathhold. After a bolus-timing acquisition to determine the delay between bolus injection and bolus arrival in the vessel of interest, a 2D gradient-echo MRA sequence is performed. Three-dimensional reconstructions can subsequently be made of the vessel, clearly depicting any region of interest. Gadolinium-enhanced MRA correlates excellently with conventional angiography (13).
A rapidly advancing technique is coronary MRA, for the depiction of the origin, course, and diameters of the coronary arteries. Congenital or acquired coronary anomalies, such as after the arterial switch operation for TGA and Kawasaki disease, can be detected without the need for the conventional catheter-based coronary angiography
(14,15). A recent MRI study showed the feasibility in patients with TGA, with clear depiction of the coronary ostia and proximal coronary arteries (Fig. 30.5). Coronary MRA combined with delayed enhancement (described next) can provide accurate insight into the myocardial perfusion status (15).
(14,15). A recent MRI study showed the feasibility in patients with TGA, with clear depiction of the coronary ostia and proximal coronary arteries (Fig. 30.5). Coronary MRA combined with delayed enhancement (described next) can provide accurate insight into the myocardial perfusion status (15).
![]() Figure 30.3. Tissue velocity MRI of the myocardium of the right ventricle. A: Example of the anatomic location of the myocardium of the RV free wall, where measurements can be performed. Region of interest (ROI; white oval also on B,D) indicates myocardial segments where tissue velocity measurements can be performed. B: Example of the anatomic location of the myocardium of the right ventricular outflow tract (RVOT) segment where measurements can be performed. C: Velocity encoded image of the four-chamber view. Velocity is encoded in the longitudinal direction. ROI is positioned at the basal RV free wall in all cardiac phases. The average velocity at the ROI is calculated in every phase and can be displayed in a time- velocity curve from which peak velocity at the RV free wall and time to peak systolic velocity at the RV free wall can be derived (E). D: Velocity encoded image of the RVOT. Velocity is encoded in the longitudinal direction. ROI is positioned at the lateral wall of the RVOT in all cardiac phases. The average velocity at the ROI is calculated in every phase and can be displayed in a time-velocity curve from which peak velocity at the RVOT and time to peak systolic velocity at the RVOT can be derived (F).Time in graphs shown as percentage of RR-interval. Ao, aorta; LA, left atrium; PA, pulmonary artery; RA, right atrium; LV, left ventricle; RV, right ventricle. (Reprinted from van der Hulst AE, Roest AA, Delgado V, et al. Corrected tetralogy of Fallot: Comparison of tissue Doppler imaging and velocity-encoded MR for assessment of performance and temporal activation of right ventricle. Radiology. 2011;260(1):88-97, with permission.) |
Delayed Enhancement Imaging
Myocardial delayed enhancement can be used to determine viable and nonviable myocardium within regions of interest. Fifteen minutes after the administration of gadolinium, the nonviable cardiac regions show hyperenhancement on
T1-weighted images compared with the normal myocardium. This technique has already been proven useful in patients with coronary artery disease, nonischemic cardiomyopathies, and myocarditis. Recent reports indicate that it can be used in CHD as well, specifically in anomalies associated with perfusion defects and congenital coronary artery anomalies (15). Delayed enhancement may be used to identify postoperative scar tissue in the right ventricular outflow tract (RVOT) in patients with TOF. Fibrosis in the RVOT appeared to be associated with RVOT dilatation, which adversely affected RV hemodynamics (16). Delayed enhancement in the RV myocardium of a patient with TGA after Mustard repair is illustrated in Figure 30.6.
T1-weighted images compared with the normal myocardium. This technique has already been proven useful in patients with coronary artery disease, nonischemic cardiomyopathies, and myocarditis. Recent reports indicate that it can be used in CHD as well, specifically in anomalies associated with perfusion defects and congenital coronary artery anomalies (15). Delayed enhancement may be used to identify postoperative scar tissue in the right ventricular outflow tract (RVOT) in patients with TOF. Fibrosis in the RVOT appeared to be associated with RVOT dilatation, which adversely affected RV hemodynamics (16). Delayed enhancement in the RV myocardium of a patient with TGA after Mustard repair is illustrated in Figure 30.6.
T1-mapping
Since with delayed enhancement imaging the inversion time is deliberately chosen to null “normal” myocardium it is not suited to assess global fibrosis and only macroscopic scarring can be visualized. With the use of contrast-enhanced T1-mapping diffuse interstitial fibrosis can be detected. In a heterogeneous group of patients with corrected CHD it was demonstrated that significantly more interstitial fibrosis was present in the myocardium of the left ventricle (LV) as compared to controls (17).
MAGNETIC RESONANCE IMAGING STRESS TESTING
Detection of cardiac dysfunction at an early stage is important to allow timely (re-)intervention when necessary. Stress testing, either pharmacologic or physical, may reveal subtle abnormal ventricular function that may not be apparent at rest (18,19 and 20). Pharmacologic stress testing using MRI has successfully been applied in the evaluation of ischemic heart disease (21), and in CHD patients (22). Physical stress testing with MRI does not require infusion of inotropes and is feasible in children and
adults with CHD (18,19 and 20). Despite certain practical problems, especially related to space limitations in the scanner when performing bicycle exercise and the induction of motion-related artifacts that might degrade image quality, the use of an MRI-compatible bicycle ergometer and a dedicated exercise protocol allows measurement of large vessel flow and biventricular function in response to exercise. For example, in a study of TOF patients after correction exercise MRI revealed that although resting EF of the right ventricle (RV) was normal in 80% of patients, the majority of 93% showed an abnormal RV response to exercise. This is most likely to be caused by longstanding volume overload because of PR (19).
adults with CHD (18,19 and 20). Despite certain practical problems, especially related to space limitations in the scanner when performing bicycle exercise and the induction of motion-related artifacts that might degrade image quality, the use of an MRI-compatible bicycle ergometer and a dedicated exercise protocol allows measurement of large vessel flow and biventricular function in response to exercise. For example, in a study of TOF patients after correction exercise MRI revealed that although resting EF of the right ventricle (RV) was normal in 80% of patients, the majority of 93% showed an abnormal RV response to exercise. This is most likely to be caused by longstanding volume overload because of PR (19).
CONTRIBUTION OF MAGNETIC RESONANCE IMAGING IN SPECIFIC ENTITIES
TETRALOGY OF FALLOT
Clinical Considerations
TOF is one of the most common types of cyanotic CHD, accounting for approximately 5.5% of all patients with CHD (23). Anterior displacement of the outflow septum is the primary defect, resulting in a VSD, overriding of the aorta, RVOT obstruction, and RV hypertrophy (24). In 1955 Lillehei et al. (25) introduced the intracardiac repair of TOF, aiming at closure of the VSD and relief of the RVOT obstruction by resection or patch placement. This surgical approach is now performed early in childhood mostly without prior placement of palliative shunts (26).
Despite good survival rates (27), residua and complications after TOF repair frequently occur. PR is a common finding after surgical repair (28). Direct quantification is possible using phase-contrast MRI in the pulmonary artery (Fig. 30.7). The resulting volume overload of the RV may be tolerated for years without signs of RV failure. However, longstanding volume overload of the RV may lead to severe RV dilatation, which is associated with biventricular dysfunction (4,29,30) and ventricular arrhythmias (31). Impaired exercise capacity can be observed with MRI during physical stress testing, showing aggravation of RV dysfunction compared with resting values (19,32). Pulmonary valve replacement (PVR) resolves PR and subsequently improves cardiac hemodynamics (Figs. 30.8 and 30.9) (33,34). PVR may lead to normalization of RV dimensions when it is performed before the RV reaches 150% of its normal size (35).
Timing of PVR remains difficult, because the expected favorable hemodynamic effects have to be weighed against the risk of repeated reoperations for homograft failure. A recent report showed a dramatic improvement of RV function as expressed by RV EF corrected for PR and the RV ESV. RV EF was calculated in this study by dividing the net pulmonary flow (derived from a pulmonary artery phase-contrast study) by the RV EDV, representing the actual efficiency of the RV (34). The background of this is that traditional RV EF does not reflect the RV systolic function in patients with PR, because loading conditions are different and may change over time (e.g., after PVR). The RV EF is not capable of demonstrating these changes, because both RV SV and RV EDV incorporate the regurgitant volume. Since RV SV is smaller than RV EDV, the effect of PR on RV SV is relatively larger than the effect on RV EDV, leading to an overestimation of the actual systolic function. Similarly, after relief of PR, the percentual decrease of RV SV is larger than the percentual decrease of RV EDV. The RV EF will subsequently decrease, whereas the actual RV systolic function improves, expressed by a decrease of RV ESV (34).
Timing of PVR remains difficult, because the expected favorable hemodynamic effects have to be weighed against the risk of repeated reoperations for homograft failure. A recent report showed a dramatic improvement of RV function as expressed by RV EF corrected for PR and the RV ESV. RV EF was calculated in this study by dividing the net pulmonary flow (derived from a pulmonary artery phase-contrast study) by the RV EDV, representing the actual efficiency of the RV (34). The background of this is that traditional RV EF does not reflect the RV systolic function in patients with PR, because loading conditions are different and may change over time (e.g., after PVR). The RV EF is not capable of demonstrating these changes, because both RV SV and RV EDV incorporate the regurgitant volume. Since RV SV is smaller than RV EDV, the effect of PR on RV SV is relatively larger than the effect on RV EDV, leading to an overestimation of the actual systolic function. Similarly, after relief of PR, the percentual decrease of RV SV is larger than the percentual decrease of RV EDV. The RV EF will subsequently decrease, whereas the actual RV systolic function improves, expressed by a decrease of RV ESV (34).
Infundibular, valvular, or supravalvular pulmonary stenosis (Fig. 30.10) can also occur, which can be depicted by contrast-enhanced MRA of the pulmonary vascular tree as well as by flow mapping across the pulmonary valve. Pulmonary stenosis may result in RV hypertrophy as an adaptive mechanism. Since there is an inverse relationship between RV mass and EF, this hypertrophy will eventually impair RV contractility (36). Furthermore, the presence of PR seems to aggravate RV dysfunction (37,38). RVOT aneurysms and fibrosis of the RVOT, which may follow after RVOT reconstruction during initial repair, also have a negative impact on RV function (16,36,38). The importance of fibrosis imaging using delayed enhancement in TOF patients was furthermore stressed by the independent relation between RV LGE score and clinical arrhythmia (39).
With MRI, LV function has been identified as an independent predictor for long-term prognosis after TOF repair as well (40,41). LV function may be hampered because of adverse RV-to-LV interaction, in which a pressure-or volumeoverloaded RV causes dysfunction of the interventricular septum (36,42).
A recently recognized feature associated with TOF is dilatation of the aortic root and related aortic regurgitation (43). This phenomenon is probably caused by previous longstanding aortic volume overload, with increased aortic flow attributable to the right-to-left shunting before repair (44). In addition to hemodynamic factors, intrinsic wall abnormalities of the aortic wall (similar to patients with Marfan syndrome) have been observed in these patients as well (45). Both an aneurysmatic aortic root and aortic regurgitation
may necessitate aortic root replacement (46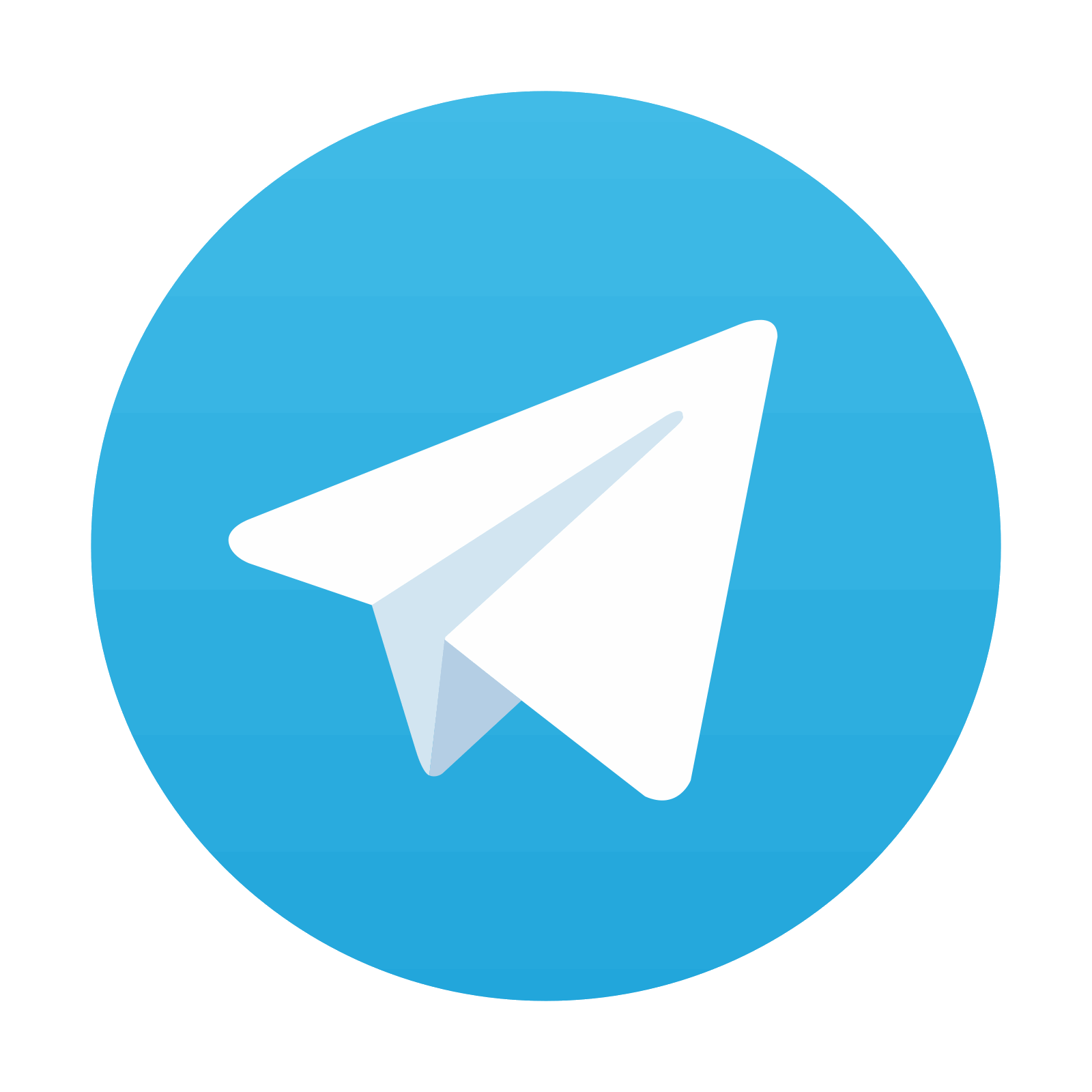
may necessitate aortic root replacement (46
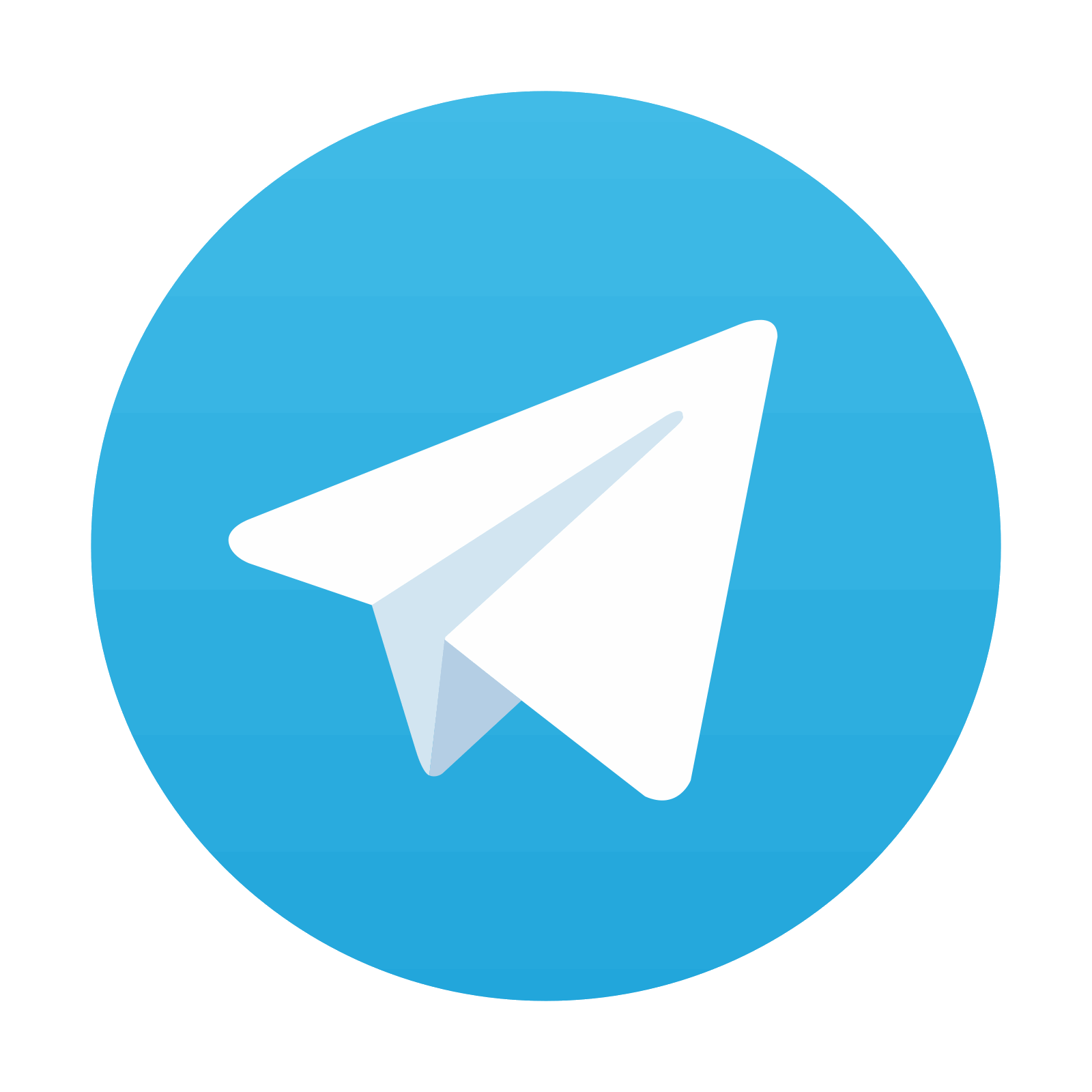
Stay updated, free articles. Join our Telegram channel
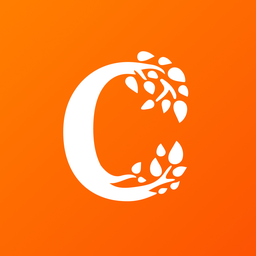
Full access? Get Clinical Tree
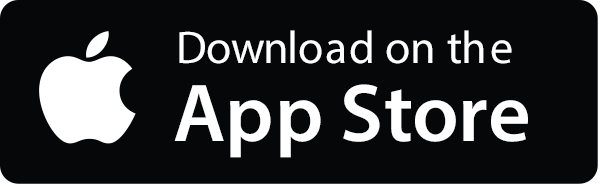
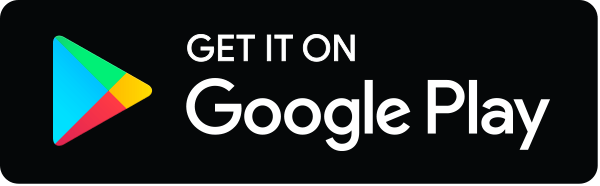
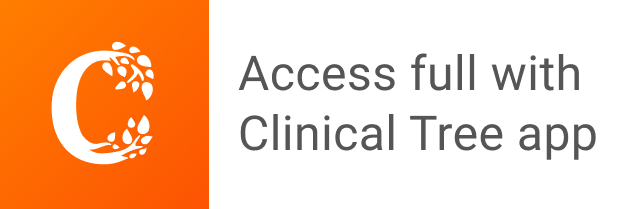