(1)
Division of Cardiology, Department of Medicine, Loyola University Medical Center, Maywood, IL, USA
(2)
Departments of Medicine (Cardiology), Radiology and Cell and Molecular Physiology, Loyola University Medical Center, 2160 S. First Avenue, Maywood, IL 60153, USA
Keywords
MR imagingMR angiographyCMRNon-contrast MRAGadoliniumPeripheral arterial diseaseClaudicationAcute limb ischemiaChronic limb ischemiaMRI safetyIntroduction
Cardiovascular magnetic resonance imaging (CMR) is routinely used in the evaluation of a wide variety of cardiovascular diseases. CMR is a noninvasive imaging modality and is widely regarded as the reference standard in the assessment of cardiovascular morphology and function. A combination of function, morphology, stress imaging for perfusion and wall motion, and late gadolinium enhancement for infarct or fibrosis imaging provides a comprehensive diagnostic evaluation by CMR. Appropriate indications for CMR have been published by the American College of Cardiology, Society of Cardiovascular Magnetic Resonance, American College of Radiology, and other relevant societies [1]. These include evaluation of LV function in heart failure or after myocardial infarction, determining viability prior to revascularization, evaluation of specific cardiomyopathies (amyloid, sarcoid, hypertrophic cardiomyopathy, arrhythmogenic right ventricular cardiomyopathy, etc.), evaluation of myocarditis or myocardial infarction with normal coronary arteries, pericardial diseases, cardiac masses, and complex congenital heart disease. There is significant ongoing research and development in CMR leading to new acquisition methods, image processing and viewing tools, and novel applications. The high spatial and temporal resolution of CMR has made it possible to investigate myocardial tissue in more detail with imaging of extracellular matrix and biologic processes at the cellular or subcellular level (molecular imaging).
Magnetic resonance angiography (MRA) of central and peripheral arteries and veins with the use of gadolinium contrast is a well-established method. Appropriate indications for vascular imaging include pulmonary vein imaging prior to radiofrequency ablation for atrial fibrillation, pulmonary artery imaging in congenital heart disease, evaluation for aortic dissection or aneurysm, diagnosis of peripheral arterial disease (PAD) , selecting patients for endovascular intervention or surgical bypass of lower extremity PAD, and post-revascularization surveillance of lower extremity PAD [1–3]. ACC/AHA 2005 Practice Guidelines for the Management of Patients with Peripheral Arterial Disease recommends that MRA of the extremities should be performed with gadolinium enhancement (class I recommendation); however, there is significant ongoing development in the field of non-contrast MRA due to the cost and safety risk of gadolinium-based contrast agents, which has shown initial promising results (see below).
CMR of heart and blood vessels is performed on clinical MRI scanners with some additional equipment. MRI scanner is a large cylindrical structure that house a powerful superconducting magnetic whose magnetic field strength is measured in Tesla (T). Commercially available MRI scanners suitable for CMR are 1.5 T or 3.0 T. Generally 3.0 T scanner provides better spatial resolution and faster acquisition speed compared to 1.5 T but may have more artifacts related to the higher field strength. Due to the technical requirements of CMR, the so-called open MRI machines are not at present suitable for cardiovascular applications. MRI images the hydrogen nuclei (1H protons) which are in high concentration in the body. Radiofrequency signals from the MRI scanner excite the 1H protons which leads to image signal generation. Special surface coils are placed over the area of interest to receive the generated signal. Images are processed and displayed on dedicated workstations. A power injector is needed to inject contrast for MRA and certain cardiac applications. A moving patient table is required to chase the contrast bolus after injection for extremity imaging. MRI-compatible patient monitoring system and infusion pump (for delivering drugs) is needed for imaging critically ill patients.
CMR has several advantages in imaging of cardiovascular system compared to other modalities. CMR provides high spatial and temporal resolution and images can be acquired in any tomographic plane without body habitus limitations. There is no ionizing radiation which makes this modality ideal for serial follow-up imaging particularly in younger patients whose radiation-related risk may be higher due to accumulated lifetime exposure. MRA is inherently three-dimensional; hence, eccentric lesions or complex vascular anatomy is better characterized to aid in procedural planning. A variety of CMR methods are used for evaluating vascular pathology. Black-blood imaging with T1-and T2-weighted techniques is used for vessel wall imaging. Vascular blood flow is evaluated by phase contrast imaging, and more recently introduced time-resolved MRA allows direct visualization of blood flow dynamics which is useful in the assessment of shunts, dissections, and conduits. There are also several specialized sequences used for non-contrast MRA that are discussed below.
This chapter provides a concise overview of contrast-enhanced and non-contrast MRA in patients with PAD. A review of MRI contrast agents and MRA techniques is also provided. For more information, readers are directed to articles referenced in bibliography and dedicated textbooks on this subject.
MRI Contrast Agents
Image contrast refers to the relative difference in signal intensity between two adjacent tissues and forms the basis for visual perception to differentiate between the two tissues in MRI. Contrast media is frequently used to enhance these differences by modifying the amplitude of the signal generated by 1H protons [4]. Gadolinium, a lanthanide metal element, is currently the most commonly used contrast agent in clinical practice. In its free form, Gd3+ is highly toxic and therefore must be complexed to a ligand or chelate before intravenous (IV) administration [5]. The chelate encapsulates the gadolinium, resulting in a thermodynamically stable and biologically inert complex.
There are currently nine available gadolinium-based contrast agents (GBCAs) approved for clinical use by the FDA in the United States. GBCAs can be further classified into categories based on their biodistribution, which is determined by the chemical structure of the chelate. The two most clinically relevant categories are the extracellular and intravascular/blood pool contrast agents.
Extracellular Contrast Agents
The first approved GBCAs were extracellular contrast agents (ECCAs ) . ECCAs distribute rapidly into both intravascular and extracellular spaces. After IV injection, the water-soluble contrast agent initially distributes into the intravascular space. Peak intravascular enhancement occurs over a short period, the arterial phase typically lasting less than 20 s in duration, followed by rapid extravasation into the interstitial space. This makes the timing of arrival of the contrast bolus with image acquisition critical. Therefore, although extracellular contrast agents are frequently used for MRA, they are not the ideal agents for this because of their pharmacokinetic profile.
Intravascular/Blood Pool Contrast Agents
Intravascular or blood pool contrast agents were specifically designed to overcome this limitation of ECCAs through deliberate confinement to the intravascular space. This was achieved by modifying the size, charge, and molecular shape of the ligand relative to the permeability of the capillary bed, resulting in a longer plasma half-life with prolonged intravascular enhancement on the order of tens of minutes as compared to seconds with ECCAs [6]. Blood pool agents therefore eliminate the need for bolus timing and allow for the imaging of multiple vascular beds, both arterial and venous phase imaging, and covering a larger anatomic area without the need for reinjection. The commercially available intravascular contrast agent is gadofosveset (Ablavar) which is FDA approved for imaging of aortoiliac disease in adults.
Novel/Experimental Contrast Agents
Ferumoxytol (Feraheme, AMAG Pharmaceuticals, Cambridge, MA) is a ultrasmall paramagnetic iron oxide nanoparticle which is FDA approved as an IV iron replacement therapy for the treatment of iron deficiency anemia in adult patients with chronic kidney disease (CKD). More recently, ferumoxytol has been investigated for use as a contrast agent in MRI [7]. Ferumoxytol has a long half-life of 14–15 h and behaves as a blood pool agent, distributing only in the intravascular compartment. Because ferumoxytol is made of iron oxide particles, it carries no risk of nephrogenic systemic fibrosis (NSF) as seen with GBCAs. Ferumoxytol has been used off-label as a MRA blood pool agent, in the imaging of the carotid arteries, thoracic and abdominal aorta, renal arteries, and peripheral arteries and veins. The main advantage is likely to be in patients with end-stage renal disease where GBCA use is contraindicated.
Safety of Gadolinium-Based Contrast Agents
GBCAs are generally considered safe with an exceptionally low incidence of adverse reactions occurring with a frequency of 0.07–2.4 % [8]. The vast majority of reactions are considered mild as defined by the American College of Radiology (ACR) . The largest assessment of GBCA safety came in 2013 in 120 million administrations which reported an incidence of severe reactions of only 0.003 % [9].
Nephrogenic Systemic Fibrosis (NSF)
NSF is a rare disorder characterized by fibrosis, most often involving the skin. In its more severe form, NSF can result in systemic fibrosis of the connective tissue, lungs, liver, and heart leading to debilitating contractures, multi-organ dysfunction, and occasionally death [10]. Treatment options are limited. In 2006, the link between GBCA exposure to this rare but potentially fatal disease was recognized [11].
The incidence of NSF primarily varies with the degree of renal insufficiency. It is estimated that patients with stage 4–5 CKD (eGFR <30 mL/min/1.73 m2) have a 1–7 % risk of developing NSF after exposure to GBCAs [8]. There have been no reported cases of NSF in patients with normal renal function to date. Other identified risk factors include acute kidney injury (AKI) accounting for 12–20 % of confirmed NSF cases, high dose GBCA [12], and multiple GBCA exposures [13].
Although the link between NSF and the exposure to GBCAs in the setting of severe renal impairment is widely recognized, the exact pathogenesis remains unknown. The leading hypothesis relates to the dissociation of gadolinium from its chelate due to prolonged elimination times in patients with impaired renal function . This has been supported by the finding that the agents most associated with NSF are the least thermodynamically stable contrast agent [14]. The data for the intravascular contrast agent gadofosveset (Ablavar) is limited.
Based on the current level of understanding, there are several measures that should be taken to minimize the risk of NSF. First and foremost, a screening method should be instituted to identify those patients at risk for NSF prior to GBCA exposure. Once a patient at risk is identified, alternative diagnostic testing should be considered. If there is no acceptable alternative and the clinical indication is compelling enough, a GBCA with a lower incidence of NSF should be used and at the lowest possible dose necessary. The institution of such simple precautions has already resulted in a profound reduction in the incidence of NSF with a near elimination of new cases [15].
Special Populations
Pregnancy
In animal models, GBCAs have been shown to cross the placenta within minutes of IV administration and, when administered at high and/or repeated doses, result in several adverse effects including fetal growth retardation, fetal loss, and teratogenicity [16]. In the limited human studies, there have been no known adverse human fetal effects after giving clinically recommended doses of GBCAs during pregnancy [17]. However, given the findings in animal studies, all FDA-approved gadolinium chelates are classified as “Pregnancy Category C” and should only be used during pregnancy when the benefits outweigh the potential fetal risks. In that event, a GBCA considered low risk for the development of NSF should be used and at the lowest possible dose necessary.
Breastfeeding
It has been shown that <0.04 % of the maternal dose is excreted into the breast milk in the first 24 h and estimated that <1 % of the contrast medium is subsequently absorbed from the infant’s gastrointestinal tract [18]. This would result in an expected systemic dose absorbed by the infant of <0.0004 % of the maternal dose, an amount far less than the permissible dose in neonates [8]. Accordingly, the current ACR position is that it is likely safe for a mother to continue breastfeeding after receiving GBCAs . Ultimately, an informed decision to abstain from breastfeeding should be left up to the mother, although there is no benefit in doing so beyond 24 h.
MRA Techniques
Contrast-Enhanced MRA (CE-MRA)
CE-MRA was first introduced in the early 1990s and represents the workhorse MR technique for imaging peripheral vasculature. CE-MRA uses GBCA, which shortens the T1 of spins in blood and is independent of flow pattern, direction, or velocity [19]. Because the arterial phase of GBCA is only 20 s, the images are acquired rapidly by arterial first pass imaging; however, newer blood pool gadolinium-based agents are available for steady-state imaging as discussed above.
CE-MRA Technique
Patient Preparation
MRA procedure must be explained to the patient in detail. If a patient has rest pain or skin ulcerations, appropriate care must be taken of the affected limb and analgesics provided if necessary. Patients must remain still during the scan, as movement will affect image quality. A 1.5 T MRI machine with high-performance gradient strength is adequate for peripheral MRA. Incremental gains in study quality can be obtained with higher gradient strengths, multichannel phased array coil, and a 3 T scanner. Dedicated bilateral lower extremity phased array coils are ideal for peripheral MRA image quality. Additional body coils will often be placed over the lower abdomen just above the extremity coil assembly to image the abdominal aorta and iliofemoral vessels. Patients are placed feet first with their arms by their sides and are forewarned that the table will move during the study and compression devices may be placed about the thighs to minimize venous contamination [20]. Care must be taken not to compress any regions especially the calves because this may result in pseudo stenosis. For lower extremity MRA, a 20 gauge venous access must be obtained in either antecubital fossa. Lower limb venous access is not recommended due to significant venous contamination of the region of interest. A multiphase MR-compatible injector pump is important in attaining consistency in injection timing and flow rates [21]. Extension tubing flushed with normal saline is required to reach the pump injector. Once a patient is appropriately positioned, large field of view localizers are obtained. In obese patients and crossover bypass grafts, it is important to ensure that relevant vascular anatomy is not excluded from the imaging volume. Rapid scout images in axial, coronal, and sagittal orientations are obtained. These initial images are useful to define the vascular territory to plan the eventual MRA examination . Individual 3D volumes oriented in the coronal plane are acquired sequentially in multiple stations (abdomen, pelvis, legs, and calves) chasing a gadolinium-based contrast agent as it flows down into the peripheral vessels (Fig. 18.1). Subtraction of the post-contrast images from the pre-contrast images is performed and the data is transmitted to a 3D workstation.


Fig. 18.1
Peripheral multi-station 3D contrast-enhanced MRA. The sketches show a longitudinal cross section of a scanner. The patient , with RF surface coils on top, is firstly positioned with the thorax in the isocenter of the scanner (a). The coronal 3D acquisition volume [three-dimensional (3D) slab] is positioned within the isocenter. During the course of the examination, the patient platform, together with the patient, is advanced stepwise toward the feet (b–d). Given the appropriate synchronization between the contrast agent bolus and the imaging time per station, the contrast agent bolus is followed along its path from the aorta via the pelvis-leg vessels to the feet. This also allows vessels to be depicted whose dimensions exceed the size of a conventional FOV (MRA in Fig. 18.2). From Kramer H et al. Eur Radiol 2008; 18:1925–1936. Reprinted with permission from Springer
MRA Acquisition
To maximize tissue contrast, the sequences are heavily T1 weighted, and acquisition is timed to the maximum intra-vascular concentration of gadolinium. Typically a fast 3D gradient echo sequence is used for imaging acquisition. Images are acquired first without contrast (mask images) and then same acquisition is repeated with contrast. Subtraction of mask images from the contrast-enhanced images suppresses the background tissue signal. The major limitation with this method is patient movement and breathing between the mask and contrasted portions of the scan and different table positions between stations, which may result in misregistration artifacts. Image acquisition (both mask and actual CE-MRA) must be obtained with breath holding, which significantly improves image quality [22].
In CE-MRA the synchronization of image acquisition to intra-arterial GBCA concentration is extremely important, and data acquisition must coincide with the arrival of the contrast in the vessel of interest. The GBCA dose volume is small, and since the acquisition time for MRA is long, the contrast bolus must encompass 40–60 % of the duration of acquisition for optimum image contrast. Improper timing of acquisition results in image artifacts that can be especially problematic with bolus chase MRA of extremities, in which multiple stations are acquired with one contrast bolus. On average, the contrast material requires 24 s to reach the femoral artery but only 5 more seconds to reach the popliteal artery and additional 7 s to reach the ankle artery [23]. The rapid transit of contrast makes it especially difficult for first pass CE-MRA as table movement and imaging time needs to be fast enough to keep up with the bolus transit. In recent years, parallel imaging (SENSE, SMASH, GRAPPA) has emerged as powerful method to decrease acquisition time without degrading spatial resolution. In parallel imaging, data are acquired simultaneously using multiple surface coils with different spatial sensitivities. The disadvantage of parallel imaging is reduced signal to noise ratio (SNR) in proportion to the acceleration factor. Due to intrinsically higher SNR, higher acceleration factors can be achieved with 3 T MRI scanners.
We typically use 0.1–0.2 mmol/kg dose of intravenous GBCA for CE-MRA at the infusion rate of 2 cc/s using a power injector followed by a saline flush of 20 cc to ensure that the contrast material does not pool within the tubing or peripheral veins and is delivered to the central veins. Since the time to peak arterial enhancement is dependent on multiple variables such as contrast injection volume and rate, saline flush volume and rate and cardiac output, it is not possible to predict the scan delay reliably; a “test bolus method” or “bolus-tracking method ” should be used to assess the peak-enhancement time.
The test bolus method measures the time taken for a small amount of contrast agent (1–2 mL) to travel from the injection site to the vessel of interest. After injection of 1–2 mL contrast, serial images of the target vessel are sequentially acquired at the rate of 1 image/s for 40–60 s. Signal intensity in the vessel is then graphically plotted against time and the time to peak enhancement is calculated. However, physiological changes in heart rate, cardiac output, and depth of breath hold may vary between the test bolus and the full bolus.
Real time bolus-tracking method: With this method visualizing contrast arrival in the vessel of interest triggers the acquisition of CE-MRA. This technique is especially useful for imaging vessels below the knees. Repetitive low resolution, background suppressed 2D images are acquired until contrast arrival and CE-MRA acquisition is triggered manually or automatically.
An important factor in achieving high diagnostic accuracy in peripheral MRA is the spatial resolution. Reliable diagnosis of arterial stenosis in MRA requires at least 3 pixels to be present across the arterial lumen. In the lower leg, this can be challenging, as the vessel diameters are 2–3 mm in this area. 3D computer workstations are used for image post-processing and viewing. The post-processing techniques used are multiplanar reformations (MPR) , curved MPR, maximum intensity projections (MIP) , and volume rendering (VR) . MPR allows viewing anatomy in all planes and the image resolution is improved if the acquisition voxels are isotropic. Curved MPR allows reformation along tortuous vessels. MIPs produce images comparable to digital subtraction angiography and can provide a quick preview of the peripheral vasculature, but diagnosis must always be confirmed by looking at thin MPR images. Volume-rendered images allow excellent display of surface-rendered 3D images but are not useful for stenosis quantitation.
Types of CE-MRA
First Pass CE-MRA
There are several types of first pass CE-MRA acquisitions based on region of interest. Single-station MRA is used to acquire a single field of view when the area of interest is limited such as the abdominal aorta, carotid arteries, and renal arteries. Multi-station MRA is used to image the peripheral vasculature from the abdominal aorta to the feet by acquiring multiple overlapping fields of views. In multi-injection MRA, contiguous overlapping stations are acquired with separate injection of GBCA at each station but are limited by the total volume of GBCA that can be administered and by the effects of residual contrast in the blood pool from the previous injections. In moving table MRA, the entire region of interest is acquired by moving the scanner table through the center of the magnet in a stepwise fashion chasing a single contrast bolus through multiple discrete stations (Figs. 18.1 and 18.2). Typically three discrete stations are imaged in peripheral MRA: aortic bifurcation and the iliac arteries, femoral arteries, and the runoff vessels (Fig. 18.3). Image acquisition must be fast enough to keep up with the arterial bolus. Failure to do so will result in incomplete arterial opacification and venous contamination that can further degrade image quality. The sequential bolus chase acquisition images the lower leg region last and is most likely to suffer from venous contamination. The most common cause of venous contamination is timing problems but may also occur as a result of inflammatory hyperemia in cellulitis or spontaneous or iatrogenic formation of arteriovenous shunts in patients with diabetes and prior surgical or endovascular interventions. Faster acquisition times decrease the likelihood of venous contamination. Biphasic contrast administration with the initial rate of 1.5 mL/s followed by 0.5 mL/s prolongs the arterial phase. The latter phase equals tissue extraction and reduces venous filling [24]. Venous compression techniques using blood pressure cuffs inflated to 50–60 mmHg around the thigh or calf have proven effective in reducing venous contamination [20]. However, cuff compression should not be used in patients with superficial vascular bypass grafts due to the risk of thrombosis. In patients with bypass grafts, hybrid protocols in which the leg station is imaged first followed by abdomen and thigh result in lesser venous contamination.



Fig. 18.2
Contrast-enhanced peripheral MRA acquired with the multi-station technique (a) compared with the “move during scan“(MDS) technique (b), with which continuous data acquisition takes place during patient table movement. The multi-station technique requires acquisition from several discrete, easy-to-overlap FOVs (a), whereas the MDS technique delivers a large, seamless FOV. From Kramer H et al. Eur Radiol 2008; 18:1925–1936. Reprinted with permission from Springer

Fig. 18.3
Peripheral CE-MRA in a patient with peripheral arterial disease . Note: occlusion of the left superficial femoral artery (arrow). In this patient, bolus-acquisition timing was successful with resulting good arterial contrast and no venous overlay. From Nielsen YW, et al. Acta Radiologica 2012;53:769–77. Reprinted with permission from Sage Publications
Steady-State MRA
Blood pool contrast agents allow us to image the peripheral vasculature beyond the short arterial first-pass phase [25]. Because there is no time constraint, MRA with very high spatial resolution can be achieved. Steady-state MRA (SS-MRA) provides images of both arteries and veins, but the excellent spatial resolution allows separation of arteries and veins. Combined first-pass and SS-MRA approach of the peripheral arteries improves results as compared to first-pass imaging alone [26].
Time-Resolved MRA
Time-resolved MRA is based on rapid repeated acquisitions of 3D images at the same location over time. Due to the rapidity of image acquisition, there is no need for appropriate contrast timing, and it provides both arterial and venous phase information in a dynamic fashion. Time-resolved acquisition techniques when used with parallel imaging can efficiently improve temporal resolution but degrade spatial resolution. Time-resolved MRA is more reliable than first-pass MRA and digital subtraction MRA for the leg station, especially in patients with arteriovenous malformations and diabetics with small vessel disease.
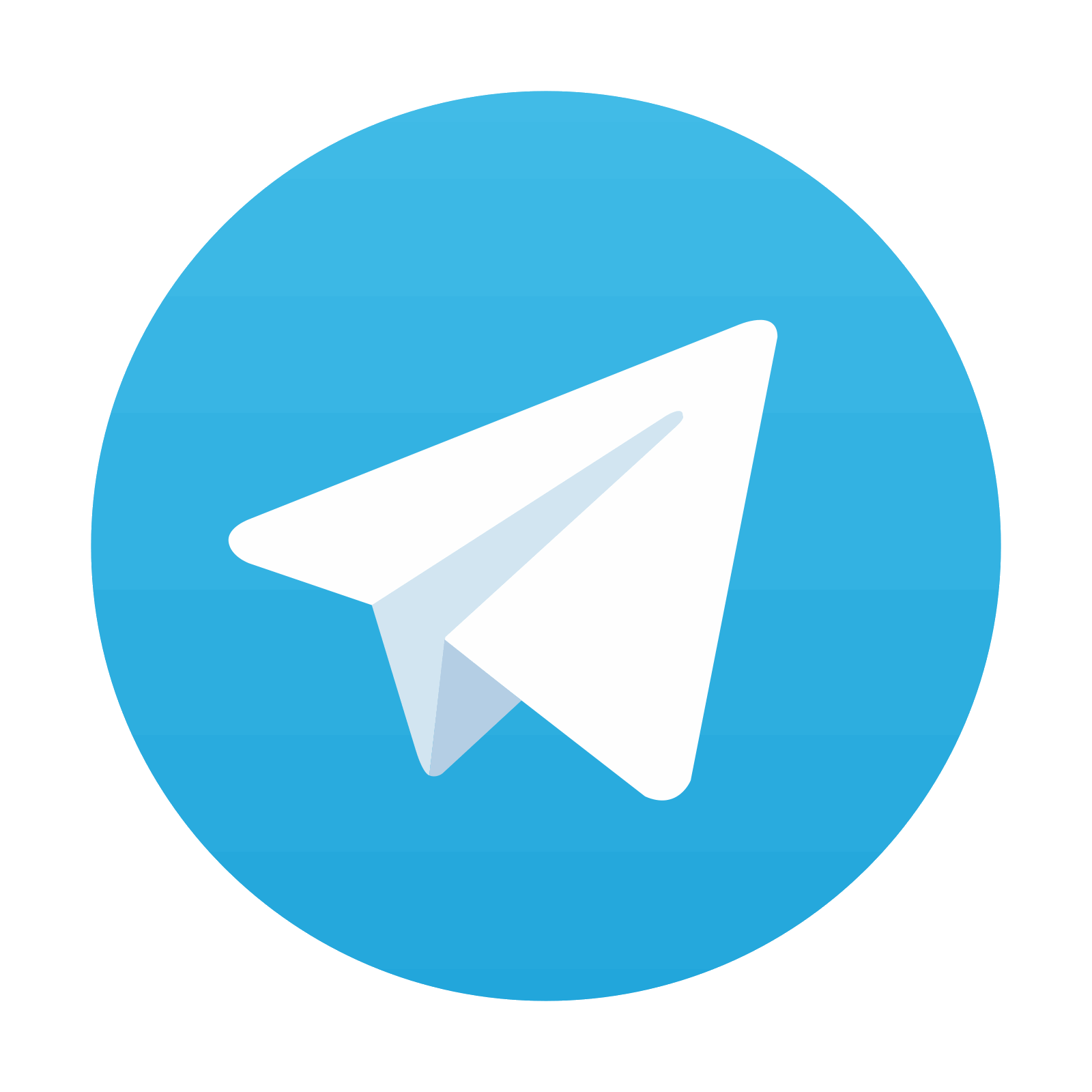
Stay updated, free articles. Join our Telegram channel
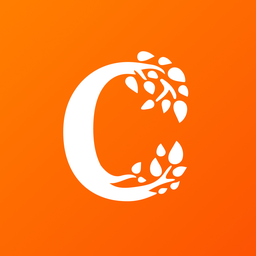
Full access? Get Clinical Tree
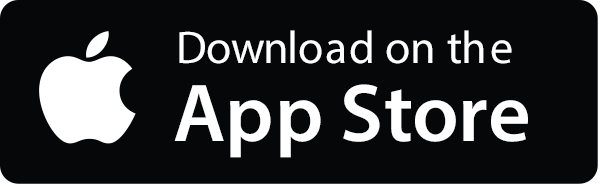
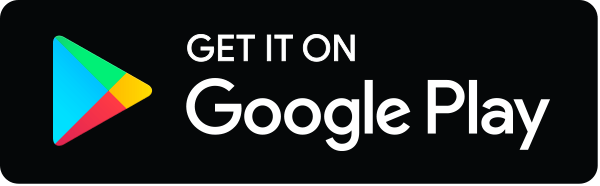
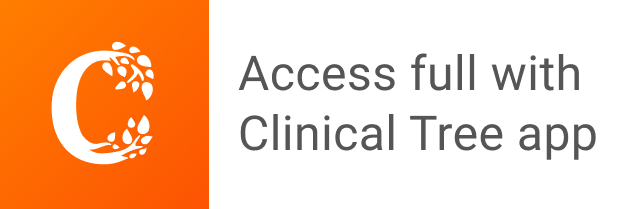