Introduction
Noninvasive cardiovascular imaging plays a fundamental role in the clinical history of patients with congenital heart diseases (CHDs) and acquired cardiac conditions, from antenatal diagnosis to long-term follow-up in adult life. Prior to birth, different noninvasive imaging modalities are used to evaluate cardiac anatomy and physiology, to plan surgical and interventional procedures, and to assess the outcomes and complications.
With a reported prevalence of 9 per 1000 live births, which corresponds to approximately 1.35 million newborns per year worldwide, CHD patients represent a very large population. Moreover, the improvement in the treatment of complex CHD has led to a substantial increase in the number of patients who reach adult age, living with a cardiac condition. In these patients, the clinical follow-up is often associated with the need for noninvasive imaging.
Most of these patients will have to undergo lifelong imaging follow-up with serial examinations, and this should be taken into account when deciding among the different imaging modalities available. The rapid technologic development of noninvasive imaging has led to a reduction in the need of cardiac catheterization.
The ideal noninvasive imaging modality should be able to assess both cardiac and extracardiac anatomy, provide information about physiology and function, and be highly reproducible, safe, and cost effective. None of the imaging modalities available can satisfy all these criteria, and therefore the assessment of children with CHD often requires a multimodality approach.
Echocardiography always represents the first line investigation when CHD is suspected and is the most common follow-up modality. It has the great advantage of being portable, rapidly available at the bedside even in emergency scenarios, but it sometimes fails to provide adequate information of extracardiac anatomy and distal vascular structures and can be limited by poor acoustic windows (e.g., after cardiac surgery). Moreover, echocardiography is less able to provide chamber quantification data for the right ventricle (RV), and this information is often crucial in patients with CHD because the RV is commonly involved.
Cardiac magnetic resonance (CMR) has emerged as a powerful technique to assess comprehensively both three-dimensional (3D) anatomy and physiology in patients with CHD. It allows accurate evaluation of both cardiac and extracardiac anatomy in multiple image planes, as well as ventricular function and physiology. Image postprocessing provides information about left ventricle (LV) and RV volumes and ejection fraction (EF) with high interobserver and intraobserver agreement, regardless of ventricular orientation and morphology. It allows quantification of valvar regurgitation, as well as stroke volume (SV) and shunt. Acquisition of 3D datasets allows 3D reconstruction of complex cardiac anatomy and evaluation of the origin and proximal course of the coronary arteries without the use of contrast media or radiation. Compared with echocardiography, CMR can add important and relevant information about cardiac anatomy, cardiac function, and tissue characterization in patients with CHD, as well as acquired cardiac conditions, summarized in Tables 21.1 and 21.2 .
Anomaly | Additional Information |
---|---|
Atrial septal defects | Assessment of associated extra cardiac defects (anomalous pulmonary venous return) Accurate quantification of shunt Accurate quantification of RV volumes and EF |
Ventricular septal defects | Assessment of location and size with possibility of 3D reconstruction Assessment of associated extracardiac defects (coarctation, patent arterial duct) Accurate quantification of shunt Accurate quantification of LV volumes |
Bicuspid aortic valve | Accurate measurements of the entire aorta Quantification of aortic regurgitation in presence of eccentric jets at echo |
Aortic coarctation | Assessment of the entire arch (hypoplasia, vascular abnormalities) Assessment of the presence of collateral vessels Follow-up after repair (recoartation, aneurysm), mostly in patient with poor acoustic window |
Patent arterial duct | Assessment of the entire arch (hypoplasia, vascular abnormalities) Assessment dimension in older patients with poor acoustic window Accurate quantification of the shunt Assessment of associated defects (coarctation) |
Anomalous pulmonary venous return | Assessment of all the pulmonary veins anatomy Assessment of associated extracardiac anomalous venous connections Quantification of the shunt Quantification of RV volumes and function |
Ebstein anomaly | Assessment of tricuspid leaflet morphology and displacement Accurate quantification of the tricuspid regurgitation Accurate quantification of the RV and arterialized RV volume and RVEF Quantification of shunt, if present |
Tetralogy of Fallot | Assessment of pulmonary regurgitation Assessment of RV volumes and EF Assessment of pulmonary arteries anatomy and flow (stenosis, split flows) Assessment of origin and proximal course of the coronary arteries |
Double-outlet right ventricle | Assessment of VSD location and commitment to great arteries Quantification of LV and RV volumes Quantification of shunt |
Transposition of the great arteries | Follow-up assessment after surgery Coronary arteries stenosis or kinking Presence of inducible myocardial perfusion defects Quantification of RV volume and function (systemic RV) |
Common arterial trunk | Anatomy of the pulmonary arteries Assessment of associated extracardiac abnormalities (coarctation) |
Anomalous origin of coronary arteries | Origin and proximal course of the LCA and RCA and relationship to the great vessels Presence of inducible myocardial perfusion defect Presence of myocardial infarction |
Single ventricle physiology | Assessment of systemic venous return before surgery (presence of bilateral SVC) Assessment of associated extracardiac abnormalities (coarctation) and collaterals Quantification of single ventricle volumes and systolic function Follow-up after Fontan operation: assessment of SVC and IVC connections to PAs, exclude presence of thrombus, assess single ventricle volume and function, quantify atrioventricular vale regurgitation. |
Anomaly | Additional Information |
---|---|
Myocarditis | Presence of myocardial edema Presence of myocardial scar (LGE) |
Kawasaki disease | Assessment of coronary artery anatomy Presence of myocardial infarction Presence of inducible myocardial perfusion defects |
Dilated cardiomyopathy | Accurate quantification of LV volumes and EF Evaluation of myocardial fibrosis (LGE) Evaluation of LV thrombi |
Hypertrophic cardiomyopathy | Accurate measurement of maximal wall thickness Evaluation of myocardial fibrosis (LGE) |
Arrhythmogenic right ventricular cardiomyopathy | Quantification of RV volumes Evaluation of RV regional wall motion abnormalities |
Muscular dystrophies | Accurate quantification of LV volumes and EF in patient with poor acoustic window |
Marfan syndrome, Loeys-Dietz syndrome | Accurate measurements of the entire aorta |
Cardiac masses | Tissue characterization Mass perfusion |
CMR also has limitations, mostly related to the long scanning time and the need for breath holds during acquisition, which cannot often be achieved properly in children. However, the development of new free-breathing CMR sequences, with faster acquisition times, will help to overcome this limitation, and CMR scanning is becoming more and more suitable for children and infants. The acquisition and interpretation of CMR images in children with CHD may be challenging and require expert physicians and technicians to obtain and interpret the images and functional information.
Cardiac computed tomography (CT) has the great advantage of providing high spatial resolution images of cardiac and extracardiac anatomy with one single, fast, free-breathing acquisition. Protocols with reduced radiation exposures have been develop for the pediatric setting; however, given the long life expectancy and the higher radiation sensitivity in children, this issue remains a consideration when choosing the optimal imaging modality.
All noninvasive imaging modalities are complementary, and it is crucial for the pediatric cardiologist to understand what each modality can offer and what are the limitations, to choose the best options for every patient, at every stage of their medical history. The aim of this chapter is to provide the clinical pediatric cardiologist with basic knowledge of image acquisition and analysis of CMR and cardiac CT.
Cardiac Magnetic Resonance
Basic Principles
Two research groups, based in Stanford and Harvard Universities, independently proposed the original notion of nuclear magnetic resonance. In 1952 Felix Bloch and Edward Purcell were jointly awarded the Nobel Prize in Physics for their development of new methods for nuclear magnetic precision measurements and associated discoveries. Almost 30 years passed before the technology became developed to create images: work that was acknowledged with a Noble Prize in Physiology/Medicine in 2003 for Peter Mansfield and Paul Lauterbur for their discoveries concerning magnetic resonance imaging.
Magnetic resonance imaging (MRI) is based on the magnetic properties and behavior of the hydrogen nuclei (protons), which are present in all body tissues. The protons, aligned in the strong magnetic field produced by the scanner magnet, can be excited by a radiofrequency pulse and change their orientation. Immediately after the radiofrequency pulse stops, the proton orientation will start to go back to the original position in the magnetic field—this phenomenon is called relaxation. The receiving coils positioned on the patient chest will detect this phenomenon and transform it into a gray scale image.
An MR sequence is given by the combination of a series of different radiofrequency pulses and transient magnetic gradients that are applied to the patient inside the scanner, in the area of the body that needs to be investigated. The timing of the radiofrequency pulses, the transient magnetic gradients, and the timing of the acquisition of the signal will determine the contrast characteristics of the image. The detailed explanation of the physics principle of CMR imaging acquisition goes beyond the purpose of this chapter, and the reader should refer to references , for a deeper understanding of CMR physics.
A CMR examination usually consists of a series of different sequences that aim to assess different aspects of the cardiac anatomy, function, and tissue characterization. It is crucial for the physician performing the CMR scan to know and understand the clinical question to decide the scanning protocol, which means the sequences that need to be acquired and their order.
Scanning children presents several technical challenges mostly related to tachycardia, the small size of the heart, and the compliance of the patients (their ability to stay still and carry out breath-holding). To overcome these issues, some adjustments are required. Fast heart rate requires high temporal resolution (20 to 60 ms) to correctly identify end-systole and end-diastole for volume quantification in cine images; this can be achieved by decreasing the number of MR data points acquired. Alternatively, in spin echo images a prospective trigger acquisition every other heart beat can be used in presence of fast heart rate. Small heart and vessel size require high spatial resolution. It is important to reduce the slice thickness and the interslice gap as much as possible and to use a small field of view.
Magnetic Resonance Imaging Protocols and Sequences
The CMR protocol is tailored for each patient, according to the cardiac defect and the clinical question. An example of a standard MR protocol for CHD can be found in Table 21.3 . Initial multiplanar localizer sequences that allow centering the heart of the patient in the scanner, to plan the following image acquisitions, are acquired at the beginning of each CMR examination followed by the rest of the protocol. The most common sequences used in children aim to assess cardiac and vascular anatomy and cardiac function and are as follows:
Sequence | Planning | Main Role | Secondary Role | |
---|---|---|---|---|
Scout/multislice | Single-shot bSSFP images | Multiple slices in all three radiologic planes | Iso-centering of the heart in the scanner | Image plane planning |
Ventricular long-axis cines (RVLA, LVLA) | Breath-held, ECG-gated, bSSFP cine images | From axial stack Place perpendicular plane through long axis of ventricle, from mid-AV valve to ventricular apex | Planning the true four-chamber image | Assessment of anterior and inferior myocardium, AV valves, ventricular sizes |
Four-chamber cine | Breath-held, ECG-gated, bSSFP cine image | From LVLA cine check that this plane passes through mid-mitral valve and LV apex From RVLA check that the plane passes through mid-tricuspid valve and RV apex | Subjective assessment of atrial volumes, biventricular volumes and function, ventricular wall motion, AV valve regurgitation | Planning short-axis stack |
Short-axis or transaxial cine stack to cover ventricles | Breath-held, ECG-gated, bSSFP cine image or breath-held real-time images | Contiguous slices placed to cover the entire ventricular mass For axial coverage plan transaxial slices from diaphragm inferiorly to outflow tracts superiorly For short-axis coverage, plan from end-diastolic frame of four-chamber cine Place image plane perpendicular to interventricular septum, and parallel to both AV valves, in both vertical long axis and four-chamber views Extend slices to include the entire basal and apical ventricular blood pool in diastolic frame | Provides the images required for segmentation of ventricular volumes | Assessment of the ventricular septum, ventricular myocardial morphology and wall motion abnormalities, outflow tracts |
Magnetic resonance angiogram | Breath-held, not ECG-gated Gadolinium injection 0.2–0.4 mL/kg Infants: injection rate 2 mL/s with 5 mL flush Older children: injection rate 3 mL/s, 10 mL flush Or time-resolved angiogram | Isotropic voxels (1.1–1.6 mm) Planned on axial HASTE stack, for coronal-orientated raw data Include anteroposterior chest wall, lung fields Image acquisition triggered with bolus tracking to ensure maximum signal in structure of interest Two acquisitions acquired, with no interval in young children, or a 15-sec interval in older children | Angiographic views of large and small thoracic vessels Images less subject to artifact caused by low velocity or turbulent flow Second-pass acquisition allows assessment of systemic and pulmonary venous anatomy | Subjective determination of preferential blood flow Can be expanded to perform time-resolved angiography or four-dimensional angiography |
3D bSSFP | Free-breathing, respiratory-navigated, ECG-gated Data acquisition optimized to occur in diastole Signal improved following gadolinium injection Signal improved in tachycardic patients by triggering acquisition with every second heartbeat | Planned axial scout images for sagittal orientation of raw data Isotropic voxels (1.1–1.6 mm) Respiratory navigator placed mid-dome of diaphragm, avoiding cardiac region of interest | Provides high-resolution images of intracardiac anatomy, including coronary arteries Allows multiplanar reformatting | Planning further imaging planes in patients with complex anatomy |
LV outflow tract cine Long-axis views through aortic arch | Breath-held, ECG-gated, bSSFP cine image | From the AV valves cine Place a perpendicular plane through both basal aortic valve and mid-mitral valve orifice Check orientation passes through LV apex using LVLA cine Cross-cut view to obtain two orthogonal cine views of LV outflow tract Plan longitudinal views of the aortic arch using a three-point planning tool from axial stack or multiplanar reformatting from 3D data | Outflow tract morphology, subjective assessment of semilunar valve function | Planning phase contrast velocity mapping Planning en face view of semilunar valve |
RV outflow tract cine Long-axis cines through branch pulmonary arteries | Breath-held, ECG-gated, bSSFP cine image | From axial stack Place perpendicular plane through the pulmonary trunk; cross-cut this view to obtain two orthogonal cine views of RV outflow tract Place perpendicular plane through the right and left pulmonary arteries, respectively, or use multiplanar reformatting from 3D data Cross-cut these views to obtain orthogonal longitudinal PA cines | Outflow tract morphology Subjective assessment of semilunar valve function | Planning phase contrast velocity mapping Planning en face view of semilunar valve |
Phase-contrast flow mapping Great artery flow | Through-plane phase contrast velocity mapping Non–breath-held, ECG-gated, phase-contrast sequence, or breath-held, prospectively triggered, spiral phase-contrast sequence | From the orthogonal outflow tract images Place a perpendicular plane across the vessel of interest Place plane just distal to valve leaflets in systole, to avoid turbulent areas of flow Optimize velocity encoding to maximize accuracy and prevent aliasing | Vessel flow volume Calculate regurgitant fractions Validate ventricular stroke volume measurements | Calculate pulmonary blood flow to systemic blood flow ratio Evaluate presence and location of shunts Calculate flow velocity |
Phase-contrast flow mapping Venous flow | Through-plane phase contrast velocity mapping Non–breath-held, ECG-gated, phase-contrast sequence, or breath-held, prospectively triggered, spiral phase-contrast sequence | From the orthogonal venous anatomy images Place a perpendicular plane across the vessel of interest Place plane between venous vessel confluence and atrial connection Velocity encoding in region 60–100 cm/s | Venous flow volume Calculate net pulmonary blood flow Calculate pulmonary arteriovenous collateral flow Calculate venovenous collateral flow | Portray sites of venous stenoses Demonstrate direction of flow in venous collateral vessels |
Phase contrast flow mapping | In-plane phase contrast velocity mapping Non–breath-held, ECG-gated | From 2D cine or multiplanar reformatted image plane Phase-encode in the predominant direction of flow | Characterize regions of flow acceleration, for CoA, PA, venous stenosis, venous baffle narrowing | Optimize through-plane flow mapping in location of highest velocity |
Spin Echo ( Fig. 21.1 )
Also known as black-blood images, spin echo can be used to assess vascular anatomy; however, because these are 2D images that often require long breath holds to acquire, they are less frequently used in day-to-day practice. They are useful for diagnosing subtle rings, flaps, and ledges within vessels or outflow tracts and for assessing changes within the vascular walls. The blood pool appears black, while the heart and the vessel wall appear bright. The acquisition is electrocardiogram-triggered and limited to a single phase of the cardiac cycle and therefore the resulting images are static. At normal heart rate the acquisition is usually set in mid-diastole, whereas at higher heart rate can be set in end-systole to minimize cardiac motion artifacts and improve image quality. The use of breath holds or respiratory navigation minimizes respiratory motion. These are among the first sequences used in CMR for CHD. Compared with other sequences, they are useful in the presence of blood flow acceleration (e.g., valve stenosis or vessel obstruction) and in the presence of metallic devices such as stents or sternal wires because they are less affected by metal artifacts.

Gradient Echo and Balanced Steady State Free Precession
In gradient echo (GRE) imaging, the blood pool appears brighter than the myocardium. The balanced steady-state free precession (bSSFP), a type of GRE, is the most commonly used sequence in CMR and can be used for cine functional and 3D anatomic imaging.
Cine images are acquired as multiple slices ideally during multiple breath holds at end expiration to minimize variations in the diaphragm position between the different slices. Retrospective ECG gating is used to allow image acquisition throughout the duration of the cardiac cycle, and the resulting images are therefore in motion. Common planes of acquisition are described in Fig. 21.2 and include vertical long axis, horizontal long axis, four-chamber, short axis, and left ventricular and right ventricular outflow tracts, although cine images can potentially be acquired in any plane. Slice thickness can be adjusted according to the structure being investigated and the patient’s size. In small children the recommended slice thickness for cine bSSFP images is 4 to 6 mm, whereas for larger children and adults the slice thickness could be set up to 8 mm. The presence of incessant arrhythmias could reduce the image quality.

Cine images provide a good contrast between the blood pool and the endocardium, allowing accurate segmentation of the cardiac chambers, and therefore are used for volumes and EF quantification ( ). Moreover, the high spatial and temporal resolution allows evaluation of myocardial thickening in systole and regional wall motion abnormalities. In patients with CHD, when quantification of RV volumes and function is a crucial aspect of decision making and clinical management, cine images represent a very powerful tool compared with echocardiography, which is not ideal for the quantification of the right cardiac chambers. The need for multiple breath holds may not be tolerated in children. Alternatively, it is possible to use real-time cine images that are performed during free breathing (see section on “ Single Shot and Real-Time Acquisitions ”). Thanks to the rapid acquisition of the data during real-time cine, the images are less affected by irregular heart rate and cardiorespiratory motion. Compared with standard breath-hold cine images, real-time cine has a lower spatial and temporal resolution, which may lead to inaccuracy in the volume measurement. To overcome this issue, new sequences have been developed with the intent to combine a rapid image acquisition with a high spatial and temporal resolution as the k-space and time sensitivity encoding imaging, which represents a useful alternative in patients for whom rapid image acquisition is desirable.
The bSSFP sequence can also be used to acquire a 3D image of the heart and great vessel that is cardiac (ECG-gated) and respiratory gated (navigator, diaphragm gated), without the need of contrast administration ( and ). It is usually acquired at end-diastole, to minimize cardiac motion, but this could be changed from patient to patient to minimize artifacts; for instance, in the presence of high heart rates, acquisition in end-systole may provide better motion compensation. It is particularly useful in patients with complex CHD because it allows analysis and reconstruction of the images in any plane, similarly to CT. The most common application of the technique is the study of the coronary artery anomalies ( Fig. 21.3 ). The main disadvantage of this sequence is the long acquisition time that can be a problem in noncompliant children.

Velocity-Encoded Phase-Contrast Images ( )
Another form of GRE imaging, velocity-encoded phase-contrast MR (PC-MR) imaging, allows for noninvasive assessment of flow in the vessels. It is the best in vivo method to quantify flow and is essential to acquire physiologic information, such as SVs, cardiac output (CO), regurgitant volumes, and shunt quantification with a good accuracy compared with invasive assessment. Flow can be measured in arteries, systemic and pulmonary veins, the aortic and pulmonary valves, and atrioventricular valves. Several technical aspects need accurate planning to avoid errors and unreliable results. The slice needs to be acquired perpendicular to the flow direction, and careful planning of the slice position is crucial to obtain reliable data. It is also important to accurately plan the maximal velocity acquired (Venc), to avoid aliasing.
Four-dimensional flow is a new 3D sequence where the three directions of flow are measured over time ( ). It enables advance postprocessing; in particular, it allows the evaluation of flows in every direction needed, without need of accurate location planning before the acquisition. It is not routinely used in clinical practice due to the long acquisition time and the need for complex postprocessing. All the flow in a selected volume of the body is acquired, and a plane can be position in any direction to visualize and quantify the flow through it. Postprocessing analysis also allows for visualization of vectors, which are a visual representation of the velocity and direction of the flow, and path lines and streamlines, which are imaginary lines that connect all the vectors through the vessel, to visually assess the flow pattern, the vortices, and the displacement of the flow ( Fig. 21.4 ). Moreover, it is used to calculate the wall shear stress, which represents the force of the flow viscosity on the endothelium of the vessel. Current research applications of 4D flow mostly study bicuspid aortic valve, aortic disease, and aortic valve interventions, but more recently the technique has been used to assess intracardiac flow in acquired and CHDs.

Contrast-Enhanced Magnetic Resonance Angiography ( Fig. 21.5 )
Contrast-enhanced MR angiography is acquired after gadolinium-based contrast administration, which increases the contrast between the blood pool and the extravascular structures. This sequence is useful to study the thoracic vasculature. It is crucial to decide carefully the timing of acquisition of the images after injection because arteries or veins will appear with greater contrast at different times. In clinical practice, a series of data sets are acquired consecutively to depict the entire thoracic vasculature in the venous and arterial phases. When reviewing and analyzing the images, it is important to remember that the reconstruction represents a mean shape throughout the cardiac cycle because the acquisition is not ECG gated. Therefore it is not possible to measure the vessel in systole or diastole. Moreover, being non-ECG gated, the images will be affected by cardiac motion and therefore intracardiac anatomy cannot be adequately investigated.

Stress Perfusion Imaging ( )
Stress perfusion MRI has limited application in children. However, it can be used to assess inducible myocardial ischemia in conditions involving the coronary arteries, such as Kawasaki disease, or when the coronary arteries have been manipulated (e.g., post arterial switch operation) and ischemia is suspected. It can be performed using either a vasodilator agent (adenosine/regadenoson) or, more rarely, an inotropic agent (dobutamine). Perfusion images are acquired at peak stress during infusion of gadolinium-based contrast media. The perfusion defects are visualized as hypointense dark regions of the myocardium that correspond to the ischemic territories. Compared with nuclear imaging techniques, adenosine stress CMR has the advantage of being radiation free and much quicker, allowing the acquisition of both stress and resting perfusion in a single examination. Careful evaluation of the patients, including medical history, medications, and ECG, is necessary prior to administration of adenosine, to screen for contraindications (asthma, allergy, conduction defects).
Tissue Characterization Sequences
Tissue characterization sequences are increasingly used in pediatric CMR. It is important to know that CMR can offer not only anatomic and functional information but also reveal insights on the myocardial tissue characteristics noninvasively. By choosing the appropriate sequences, myocardial edema, scarring, fibrosis, iron, and lipid overload can be investigated. These sequences are particularly useful also in the assessment of cardiac masses and cardiomyopathies.
T2 Short Tau Inversion Recovery.
Precontrast T2-weighted images are used to evaluate the presence of myocardial edema. These sequences can be useful in the context of acute myocarditis and myocardial infarction and in the assessment of cardiac masses.
T2* (Star).
This is a noncontrast sequence that allows for quantification of myocardial and hepatic iron overload in iron-loading conditions. It consists in the acquisition of a single midventricular short-axis slice and a single axial slice of the liver. It is recommended to perform the analysis of T2* by drawing a region of interest on the interventricular septum to minimize artifacts. Serial T2* examinations are used to assess the progression of the iron overload in the myocardium and liver and to guide therapy in conditions such as thalassemia major.
T1 Mapping and Extracellular Volume.
These novel techniques are used to evaluate the presence of diffuse interstitial fibrosis and generally to assess whether there is an increase of the volume of the extracellular space. The most common CMR sequences used are MOLLI and ShMOLLI. Native T1 time is an intrinsic characteristic of every tissue. Increases in the extracellular compartment lead to an increase of native T1 values. This can typically happen in myocardial edema, diffuse fibrosis, or protein deposition (like in amyloidosis). In contrast, the accumulation of iron and lipids tends to shorten the native T1 values. Native T1 mapping is a useful tool in investigating patients with LV hypertrophy when Anderson-Fabry disease is suspected.
Late Gadolinium Enhancement Imaging.
Late gadolinium enhancement (LGE) images should be acquired ideally 5 to 20 minutes after contrast administration. The gadolinium-based contrast agents tend to accumulate in the extracellular space, whereas they have a rapid washout from the normal myocardium. The areas in which the gadolinium accumulates will appear bright (enhanced), compared with the normal myocardium that appears black. Pathologic processes that lead to an increase of the extracellular space (myocardial scar or fibrosis) will present LGE. The pattern of distribution of the LGE follows the underlying pathophysiologic process, being full thickness or subendocardial when secondary to diseases affecting the coronary arteries and intramyocardial or subendocardial in nonischemic conditions ( Fig. 21.6 ). Although the role of LGE in adults with acquired and CHD has been well established, the applications in children remain to be fully established.

In the follow-up of patients who underwent surgery for CHD, LGE can be observed in the areas involved in the surgical intervention (apical vent, patch, ventriculotomy); areas of ischemic LGE can also be observed after surgery if small ischemic embolic events occurred during or after surgery. Several studies have evaluated the presence of LGE after surgical repair in CHD. For example, it was rarely found in adolescents after the arterial switch, whereas in patients late after Fontan operation it is associated with reduced ventricular systolic function and higher prevalence of nonsustained ventricular arrhythmias. In cardiomyopathies, LGE can be observed in children and adolescents with hypertrophic cardiomyopathy, dilated cardiomyopathy, and myocarditis.
Single Shot and Real-Time Acquisitions ( )
A completely different approach to cardiorespiratory motion suppression (ECG gating, breath-holding and navigator, diaphragm respiratory gating) is used to significantly speed up MRI acquisition into a single R-R interval—this technique is known as a single shot acquisition. This is accomplished by lowering the spatial resolution, and most single shot imaging is performed at much lower spatial resolution than gated MRI. However, techniques such as partial Fourier and dynamic k-t methods can be used to increase resolution and, along with spiral and radial MRI techniques, can be used to acquire high-quality images in as little as 30 ms. As with cardiac gated sequences, single shot imaging can be performed as a single-phase or multiphase technique. Single-phase single shot techniques are used for morphologic imaging when breath-holding is not possible. They are usually triggered to a certain part of the cardiac cycle, although this is not a necessity. Examples are scout imaging, single shot LGE imaging, and half-Fourier acquisition single-shot turbo spin echo (HASTE) imaging. If a single shot technique is continuously run, it becomes real-time imaging. Real-time MRI is still relatively underutilized in CMR; however, it does have the benefit of not requiring cardiac or respiratory gating. Its main uses have been in assessing cardiac function and flow in patients in whom breath-holding is difficult. In the future, better reconstruction algorithms may make real-time imaging the standard for CMR.
Postprocessing
After data acquisition, images are analyzed on workstations with dedicated software. Depending on the clinical question and the sequences acquired, images may require different analyses.
The most common analysis performed is the quantification of the cardiac volumes and cardiac mass ( ). This is done by drawing the endocardial contour of the LV or RV in diastole and systole from short-axis cine images ( Fig. 21.7 ) or axial imaging. A disk summation algorithm allows the software to automatically calculate end-diastolic volume, end-systolic volume, ventricular SV, and EF by computing the volume of each slice. By contouring the epicardium, mass can be calculated. Normal values for chamber quantification and vessel measurements are available for children.

PC-MR is the sequence used for evaluating cardiac and extracardiac flows ( ). Assessment of flow is performed by drawing a region of interest in the vessel being investigated and propagating it through the cardiac cycle. The blood flow through the slice will be computed, and a flow curve will be calculated. Forward flow, backward flow, and regurgitant fraction (RF) and CO are easily derived from flow analysis. Flow analysis represents an important step in the postprocessing and reporting of MRI in patients with CHD. As explained in the following paragraph, it allows quantification of cardiac shunts, regurgitation, and split flows.
Quantification of Physiology
Stroke Volume
SV is the amount of blood pumped out by the LV or RV at each systolic contraction in milliliter per beat. SV is an important physiologic parameter, and it is easily assessed by CMR from cine images with the following formula:
SV=EDV−ESV
The amount of blood pumped by each ventricle should be similar in normal hearts, and so the LV SV and the RV SV should be equal. In the presence of valvar regurgitations or shunts, the LV and RV SVs differ. Moreover, the ventricular SV should match the forward flow of the corresponding great vessel flow (aorta for the LV and pulmonary trunk for the RV, in a VA concordant hearts), in absence of atrioventricular valve regurgitation.
By multiplying the SV by the patient’s heart rate, CO can be calculated (L/min). It represents the amount of blood pumped by the investigated cardiac chamber (LV or RV) in 1 minute:
CO=SV(mL)×heart rate(bpm)
Ejection Fraction
LV and RV EF express the systolic function of the ventricles. EF can be easily calculated in CMR by analyzing short-axis or long-axis cine images as described in the previous section, using the following formula:
FE(%)=SV×100EDV
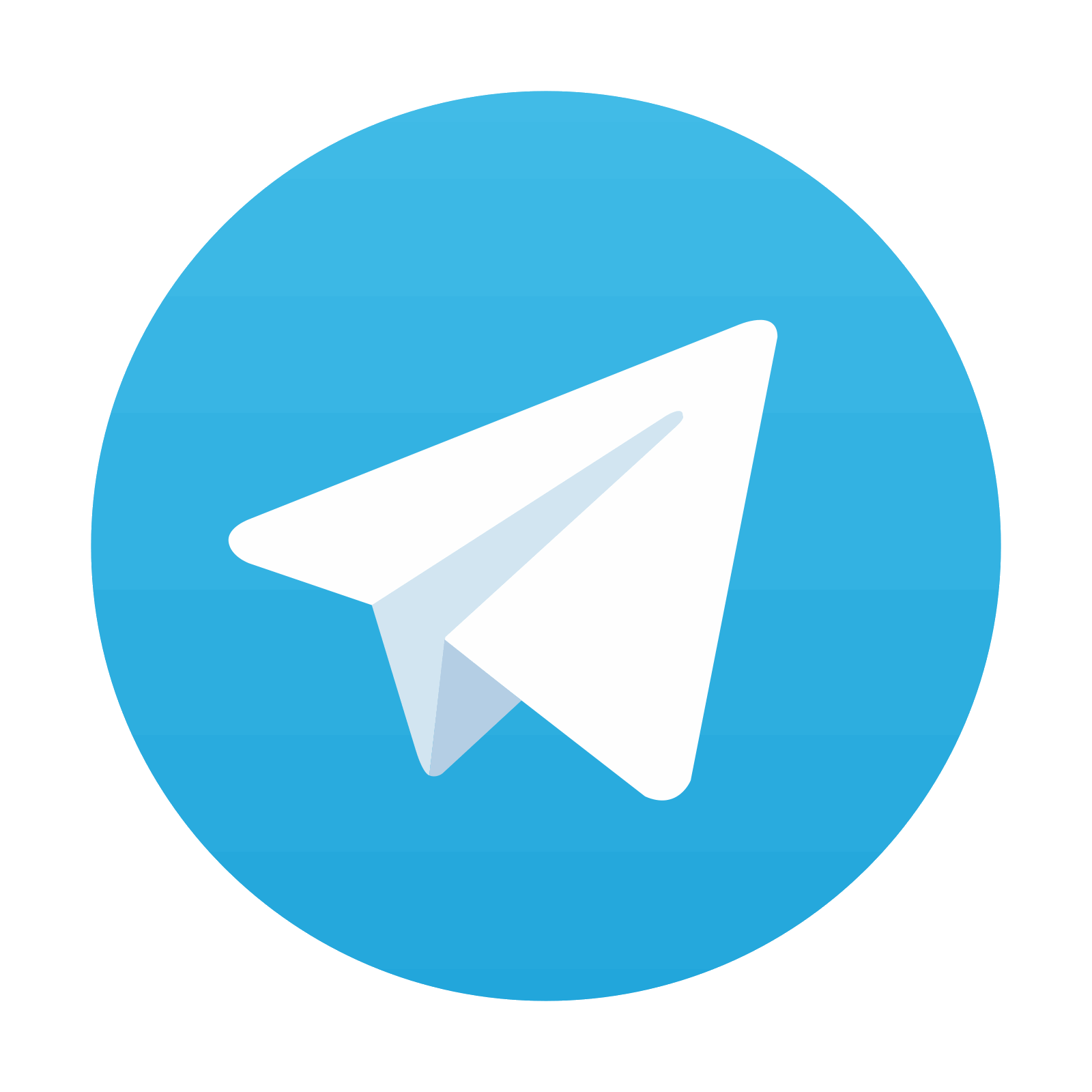
Stay updated, free articles. Join our Telegram channel
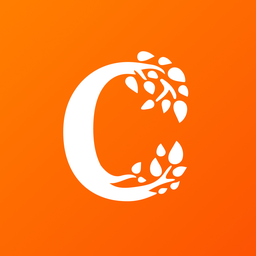
Full access? Get Clinical Tree
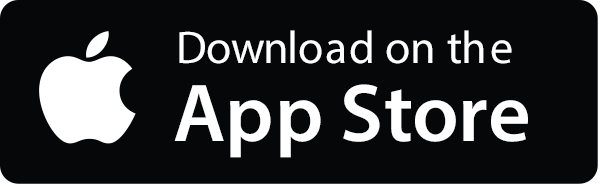
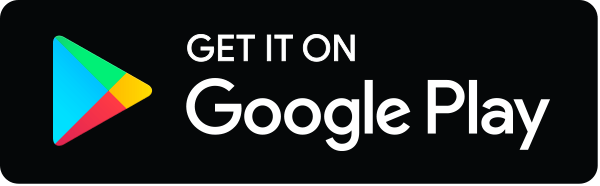
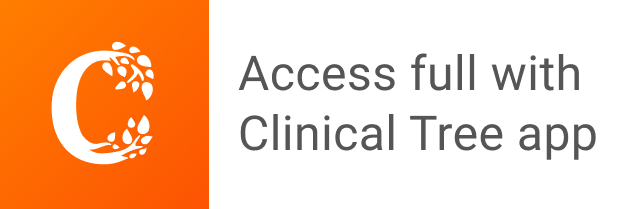