Echocardiography remains the most widely used diagnostic imaging modality in the assessment of patients with congenitally malformed hearts, owing to its portability and excellent temporal resolution, and the contrast provided by the interface between the blood and the tissues. 1 Ultrasound, however, does depend on acoustic windows, which are often limited in older patients or following surgery. Both computed tomography and magnetic resonance imaging allow imaging at all angles, and largely irrespective of surrounding structures or air. Consequently, and because they deliver multi-slice or true three-dimensional images, magnetic resonance ( Table 18D-1 ) and computed tomography help clarify the three-dimensional morphology of the cardiovascular system and its topographic relationships to the extracardiac structures, such as the trachea and bronchuses.
Post-operative tetralogy of Fallot |
Stenosis of the right and left pulmonary arteries |
Native and repaired coarctation of the aorta |
Aortic valvar insufficiency |
Marfan syndrome |
Partially anomalous pulmonary venous connection |
Functionally univentricular circulations after the bidirectional cavopulmonary connection, or with Fontan-type anatomy |
Arrhythmogenic right ventricular cardiomyopathy |
Dilated and hypertrophic cardiomyopathy |
Cardiac mass |
Takayasu’s arteritis |
The history of magnetic resonance imaging is dotted with Nobel prizes awarded in physics, chemistry, and medicine. In 1946, Felix Bloch 2 and Edward Purcell 3 , in the same journal issue but independently of one another, described the behaviour of the nucleuses of certain elements of the periodic system when introduced into a magnetic field. Imaging using magnetic resonance, however, was not possible until Paul Lauterbur applied gradients spatially to encode the signal, producing the first magnetic resonance image of two tubes of water in 1971. This work was published in 1973, 4 and, in the following year, he published the first images of a living animal, a clam. 5 When Richard Ernst applied the Fourier transformation to the technique pioneered by Lauterbur, magnetic resonance imaging, as we know it today, was born. 6 The first magnetic resonance images of the heart date back to the early 1980s. 7 Major breakthroughs include electrocardiographic gating in 1984, 8 phase velocity mapping in the same year to measure the flow of blood, 9 and gadolinium-enhanced magnetic resonance angiography in 1988. 10
Since then, improvements of the hardware, particularly stronger and faster gradients, as well as higher resolution coils, paralleled by exponential increments in the capacity to process data, have laid the foundation for an array of sequences and applications. Between the early days of cardiovascular magnetic resonance imaging in the 1980s and the present, the technique has undergone an evolution from a non-invasive anatomical tool to a powerful window towards haemodynamics and myocardial mechanics.
The technological advancement in computed tomography is moving towards faster scanning, as well as a reduction in irradiation. Both improvements have helped to establish computed tomography in the work-up of pathology of the vessels and the airways in children. We can now obtain images during spontaneous breathing, and at an acceptable level of exposure to irradiation.
In this section of the chapter devoted to imaging, we offer an overview of the information now provided by magnetic resonance and computed tomography in children with congenital and acquired cardiac disease, discussing the limitations and risks of the techniques. We hope to guide cardiologists in using the appropriate cross sectional modality for imaging their patients, and in understanding the results.
MAGNETIC RESONANCE IMAGING
Physical Principle
Magnetic resonance imaging utilises the varying magnetic properties of hydrogen nucleuses, or protons, in different tissues in the body. 11,12 When excited by a radio-frequency pulse, the protons, like small stab magnets, briefly change orientation before returning to their initial state. During this relaxation, the protons give off energy. This process is termed resonance, or echoing. It can be measured, and then translated mathematically into an image of grey values. The way these echoes are created and manipulated determines the shading of the resulting image, a process termed weighting. Manipulation of the echo can be achieved by applying one or more additional radio-frequency pulses following the initial pulse that change the spin of the protons. This is called spin echo. Another way to influence the echo is by alternating the orientation of the magnetic field of the scanner during proton relaxation, resulting in a gradient echo sequence.
Magnetic resonance imaging operates between the poles of the duration of the scan, its temporal and spatial resolutions, and the intensity of the signal, or its ratio to the produced noise. As a general rule, improving one of these features typically worsens the other three. The settings used, therefore, have to be composed to maximise the benefit from a particular sequence in each individual patient. A comprehensive approach to the physics is beyond the scope of this chapter, and the interested reader is directed elsewhere. 11
Practical Considerations and Patient Safety
Cardiovascular magnetic resonance is a complex modality. Awareness of the underlying physical principles and computations is a prerequisite to its successful application to children, in whom a cookbook approach frequently fails. The field of congenital cardiac disease, on the other hand, bears its own intricacy that must be understood to best design the examination. Training in both aspects of paediatric cardiovascular magnetic resonance is essential to maximise the gain from this technique. An intimate cooperation between radiologists and cardiologists is advocated to master and help advance the fast evolving field.
It is our practice to sedate or anaesthetise most patients younger than 6 years of age. This, however, is only a rule of thumb, and varies according to the maturity of the patient, as well as to anaesthetic support, the monitoring available, and the presence of a physician. Careful preparation and guidance of the child prior to and during the scan have a beneficial effect on the quality of the images obtained. Prior to any study, absolute and relative contraindications must be addressed. 13,14 Pacemakers and other electronic implants, such as pumps for infusing drugs, are currently absolute contra-indications to magnetic resonance imaging. Controversy surrounds magnetic resonance imaging at 1.5 Tesla following implantation of metallic objects. 15 Patients with non-ferromagnetic foreign bodies can probably undergo testing immediately after implantation. For an implant or device that is weakly ferromagnetic, such as most stents, coils, and artificial valves, it is customary to wait until at least 6 weeks after implantation in order to prevent dislodging. 14 A strong magnetic field can induce an electrical current in temporary or permanent pacing wires, causing thermal injuries to surrounding structures. 16 If they are within or close to the field of view, all objects are desirably not only magnetic resonance imaging safe but also compatible, in order not to impair the information that can be obtained from the study ( Fig. 18D-1 ). 14 If possible, dental braces should be removed. The classification into safe and compatible is currently being replaced by the terminology safe and conditional, introduced by the American Society for Testing and Materials International. According to the new terminology, a patient with an implant labeled conditional may undergo magnetic resonance testing if certain requirements pertaining to the scanner and sequences used are met. Both classifi cations currently co-exist and can be found on the device packaging. Gadolinium-based contrast mediums are safe, with a risk of anaphylactic reactions in the range of 0.001% to 0.01%. 14 Patients with severe renal insufficiency, expressed by a glomerular filtration rate of less than 30 mL/min/1.73 m 2 , face a risk of developing nephrogenic systemic fibrosis after exposure to gadolinium, and should not receive contrast. 17 Nephrogenic systemic fibrosis was initially observed in, and thought solely to affect, the skin, but internal organs such as liver, lungs, and heart may be involved. An extensive review of the safety aspects of magnetic resonance can be found elsewhere. 14 With the exception of real-time imaging and contrast-enhanced angiography, most sequences require electrocardiographic gating to compensate for cardiac motion. Respiratory motion is countered either by breath-holding, tracking the motion of the diaphragm, or acquiring multiple sets of data to average out the effects of the inconsistent position of the thorax.

Techniques for Imaging
Each examination begins with a series of static scout images, or localizers, of the thorax and adjacent body parts in three orthogonal body planes ( Fig. 18D-2 ). With most recent scanners, the scout images with an acceptable spatial resolution can be obtained within 1 or 2 minutes, making most anatomical information readily available. All subsequent sequences for detailed examination are planned using this scout, as well as any subsequently obtained images, as a reference. As a principle of prescription, any imaging plane is defined unequivocally either by three points, so-called three-point planning, or by how it dissects two separate images previously obtained, the so-called double-oblique technique.

Cine Imaging
These moving images of the beating heart are the workhorse of current magnetic resonance imaging in patients with congenitally malformed hearts ( Figs. 18D-3 and 18D-4 ; see also Fig. 18D-2 ). Blood is signal-intense, and contrasts well with the grey myocardium. Turbulent flow causes loss of signal from dephasing, and can be identified as dark streaks within the bright blood pool (see Fig. 18D-2 ). As an important difference to echocardiography, these moving images, with few exceptions, are not acquired in real time, but are assembled over many cardiac cycles, so that a temporal correlation of cardiac events with the coinciding beat-to-beat electrocardiogram is not possible. A representative work flow for cine imaging is shown in Figure 18D-3 . The strategies to achieve the basic views vary between users. A stack of 10 to 12 short-axis cine images (see Figs. 18D-3 and 18D-4 ) are used to calculate ventricular volumes and myocardial masses (see Fig. 18D-4 B). As opposed to echocardiography, this method does not rely on geometric assumptions, and is widely accepted as the gold standard tool for ventricular volumetry.


A modification of cine imaging is myocardial tagging ( Fig. 18D-5 ): Here, selective saturation pre-pulses spoil all spins within multiple planes perpendicular to the plane of imaging, thus superimposing a grid of black lines across the field of view. The black lines persist through systole, and are deformed by myocardial contraction. From the degree of deformation, it is possible to calculate radial and circumferential strain, as well as ventricular twisting. 18–21 The assessment of cardiac function is fuelled by the expectation of better understanding of myocardial mechanics, and by the hope of detecting and treating myocardial disease in its early stages. The sensitivity of analysis of regional wall motion can be increased by protocols inducing stress, using dobutamine or magnetic resonance compatible ergometers.

Measurements of Flow
Phase contrast imaging is another key technique in the assessment of congenitally malformed hearts by magnetic resonance. It is used to quantify the velocity and volumes of flow ( Fig. 18D-6 ). 22–24 The ability to assess the flow in all major vessels in any orientation makes this technique ideal for the assessment of congenitally malformed hearts. Data is acquired during spontaneous breathing or with the patient ventilated, as breath-holding manoeuvres can affect the flow of blood. The vessel is imaged perpendicular to its long axis, so-called through-plane imaging. In Figure 18D-7 ,we show the prescription of the planes to image the great vessels as well as the atrioventricular valves, using the double oblique technique. Phase contrast sequences produce a cine modulus image (see Fig. 18D-6 A) of the anatomy, as well as a velocity-encoded image which contains the information pertaining to flow (see Fig. 18D-6 B). Phase contrast imaging is used to quantify valvar regurgitation. Flow can accurately be measured in the right and left pulmonary arteries, as well as in systemic vessels. By subtracting pulmonary arterial influx from venous efflux, the amount of systemic-to-pulmonary arterial collateral flow can be calculated in patients with a functionally univentricular circulation, or in those with obstructed right ventricular outflow tracts. 25–27 Flow across a patent arterial duct can be directly measured. Intracardiac shunts can be calculated by comparing flows in the ascending aorta and pulmonary trunk. 28,29 Unlike ultrasound Doppler, phase contrast imaging allows analysis of the pattern of flow in all vessels shown in the field of view, helping clarify timing of flows relative to one another. Certain conditions exhibit characteristic patterns: for example, the configuration of the velocity of flow in patients with pulmonary hypertension ( Fig. 18D-8 ) is characterised by an early systolic peak with decreased maximum velocity; one or more secondary peaks; early cessation of systolic forward flow, with a nadir arriving before that of the ascending aortic flow; and undulation of velocities during diastole. 30 Flow trajectories and turbulence can be assessed by imaging along the vessel or jet of flow, known as in-plane imaging, and, experimentally, it is possible to produce four-dimensional phase contrast imaging ( Fig. 18D-9 ). A number of conditions compound the accuracy of phase contrast magnetic resonance, including insufficient temporal resolution, a too high or too low limit of encoding, as well as non-laminar target flow. 23 Large translational movements of the great arteries through the fixed imaging plane, as well as turbulence and swirling in frequently dilated arterial roots hamper the quantification of regurgitation across arterial valves. 31,32




Contrast-enhanced Magnetic Resonance Angiography
Gadolinium-based contrast agents can be used to increase the signal from the blood and reduce the time required to acquire images, thus allowing three-dimensional angiography with a submillimeter spatial resolution ( Figs. 18D-10 to 18D-12 ; see also Fig. 18D-9 ). When repeated in short intervals, four-dimensional angiography can be performed, with time added as the fourth dimension to the three-dimensional anatomy. The practical temporal resolution of four-dimensional or time-resolved magnetic resonance angiography for the pulmonary vasculature is between 0.5 and 1.0 seconds, depending on the imaging volume and the spatial resolution. The interpretation of an angiogram should always begin with a careful review of the source data. Image processing can highlight the information contained in the images, clarify topographic relationships, and facilitate demonstration of the anatomy to cardiologists and surgeons. A three-dimensional data set can be displayed either as a projection or as a volume rendered image ( Fig. 18D-13 ; see also Figs. 18D-10 through 18D-12 ). Maximum intensity projections (see Figs. 18D-12A and 18D-13A ), the most commonly used type of projection, resemble static images from fluoroscopic angiography. To create these images, the computer retains the brightest voxels along a virtual beam of projection. The orientation of this projection, as well as the thickness of the projected slice, is freely adjustable during post-processing. Computationally intensive volume rendered images (see Figs. 18D-10, 18D-11, 18D-12B, and 18D-13B ) lend a plastic appearance to the anatomy, optionally including colour and a virtual source of light.



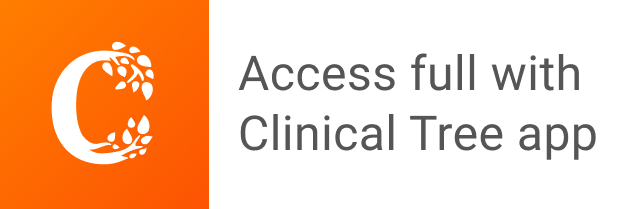