Introduction
The ability to accurately measure blood flow and oxygen saturations across the human late-gestation fetal circulation offered by advances in magnetic resonance imaging (MRI) has provided new insights into the impact of congenital heart disease (CHD) on the developing fetus. MRI has demonstrated redistribution of blood flow and interruption of the normal streaming of blood resulting from the obstructions to flow and abnormal connections that characterize CHD. Abnormal fetal hemodynamics have been linked to the dysmaturation of the lungs and brain that are typical of newborns with more severe forms of CHD. We have also learned how diminished cardiac output in fetuses with single-ventricle physiology is associated with reduced fetal perfusion of the placenta and impaired placental oxygen exchange, which may account for the growth restriction typical of late-gestation fetuses with these types of cardiac malformations. Recent data indicate that an impaired maternal-fetal environment may have an important impact on congenital cardiac surgical results. These observations are likely to be of relevance as we seek to understand better the risk factors for adverse outcomes of neonatal cardiac surgery and develop new therapies to alter the natural history of CHD prior to birth.
Normal Fetal Circulatory Physiology
Much of what is known about fetal cardiovascular physiology has been learned through invasive experiments performed in sheep. Elaborating on methods for exteriorizing and catheterizing the fetal lamb developed by Joseph Barcroft and Geoffrey Dawes at Cambridge and Oxford universities, Abraham Rudolph and his coinvestigators at the Cardiovascular Research Institute at the University of California, San Francisco, defined our modern understanding of the distribution of blood flow and oxygen transport across the fetal circulation. Rudolph’s flow measurements were achieved through the selective injection of radioactive microspheres into different venous compartments that were subsequently trapped in the microcirculation of the end organs supplied by the fetal heart. By measuring the relative activity of the different tracers in each of the various fetal organs and applying the Fick principle to measure umbilical flow, blood flow in each of the major vessels was calculated. These flow measurements were combined with blood pressure measurements and oximetry performed by conventional blood gas analysis of samples obtained using catheters placed in the fetal vessels. Based on observations regarding differences between the species, including the size of the fetal brain and hematocrit, Rudolph then predicted the distribution of blood flow and oxygen saturations across the human fetal circulation. To summarize, the fetal circulation operates in parallel, with shunts at the ductus venosus, foramen ovale, and ductus arteriosus, which allow well-oxygenated blood returning from the placenta to pass directly to the most metabolically active fetal organs, the heart and brain, while deoxygenated blood is directed back to the placenta. In the fetus, the blood with the highest oxygen saturation of approximately 85% is found in the umbilical vein and, downstream, the oxygen saturation is 10% to 15% higher in the left heart (65%) than the right (55%) (see ). In fetal sheep, this is achieved by a remarkable streaming of blood emerging from the ductus venosus and left hepatic vein toward the foramen ovale, while more deoxygenated blood passes from the infrahepatic inferior vena cava and right hepatic vein toward the tricuspid valve.
In fetal sheep, the right ventricle provides the more dominant contribution (approximately two-thirds) toward the combined ventricular output, the majority of which passes into the ductus arteriosus and descending aorta. Rudolph suggested that the right ventricle was also dominant in the human, with about 15% of the combined ventricular output passing into the pulmonary circulation, which remains vasoconstricted in lambs because of the low oxygen saturation of the blood supplied to the pulmonary arteries. Of the left ventricular output, the majority passes into the head and upper limbs, returning to the right atrium via the superior vena cava (SVC), while a small proportion passes across the aortic isthmus to join the ductus arteriosus flow in the descending aorta. Rudolph estimated that descending aortic flow accounted for approximately 40% of the combined ventricular output in the human fetus, with 25% being returned to the placenta via the umbilical veins. Foramen ovale flow was calculated to comprise 20% of the combined ventricular output, with 3% being supplied to the coronary circulation. Similar estimates of the distribution of the normal fetal circulation have subsequently been reported based on ultrasound measurements of blood flow made in human fetuses. However, the measurement of fetal vessel flow by ultrasound is challenging due to difficulties in accounting for variations in flow velocity across the vessel lumen, problems with making accurate vessel area measurements, and limitations in obtaining an adequate angle of insonation. These limitations have resulted in limited data regarding the redistribution of flow that is expected to result from CHD in the fetus. Instead, investigators have focused on other Doppler ultrasound measures, such as peak velocity or pulsatility index, to assess the impact of obstructions to flow or changes in downstream vascular resistance to help interpret fetal cardiovascular physiology in human fetuses with CHD. Similarly, changes in fetal oxygenation have been inferred from Doppler assessments of changes in placental and cerebral vascular resistance, and so such data also have major limitations.
Magnetic Resonance Imaging Techniques for Assessing Fetal Circulatory Physiology
While technical challenges arising from artifacts resulting from fetal motion and difficulties in obtaining adequate signal from small blood vessels remain, noninvasive MRI techniques for use in human pregnancies have recently been developed. The data from these studies resemble the invasive flow and oximetry measurements that Rudolph first reported in fetal sheep 50 years ago. This approach is currently only feasible in late-gestation fetuses and consists of cine phase contrast vessel flow measurements and oximetry based on magnetic resonance (MR) relaxometry.
Cine Phase Contrast Magnetic Resonance Imaging Flow Quantification
As the electrocardiographic signal usually used for temporal calibration of cardiac MRI is not easily available from the fetus, alternative methods for achieving high-resolution flow measurements have been developed. Metric optimized gating is a technique that uses a postprocessing solution to achieve cardiac triggering. To summarize, the MRI data are acquired using an artificial QRS complex, which has been programmed into the imaging acquisition, as shown in Fig. 7.1 . The data are then reconstructed through a range of candidate heart rates, with the heart rate that was present during that particular acquisition identified through the lack of artifact in the resulting images. Examples of cine phase contrast acquisitions obtained in the ascending aorta at 1.5 and 3.0 T using metric optimized gating are shown in Fig. 7.2 , which demonstrates the improvement in signal-to-noise ratio with higher magnetic field strength. The imaging plane is obtained in the short axis of the vessel, allowing for “through plane” quantification of flow. The accuracy of the flow measurement depends on achieving a true short-axis orientation, which can be achieved by obtaining two orthogonal long-axis views using anatomic survey images through the thorax and upper abdomen. Following metric optimization of the phase contrast acquisition, a region of interest is placed around the vessel using standard postprocessing software in order to quantify flow, which can be indexed to fetal volume (or weight) based on segmentation of the fetal envelope. When acquiring phase contrast flow imaging it is important to have adequate spatial resolution, which entails providing at least 8 voxels across the vessel cross-section, and adequate temporal resolution—in the range of 50 ms or better.


Magnetic Resonance Oximetry
MR oximetry exploits the different magnetic properties of oxygenated and deoxygenated hemoglobin, whereby the transverse or T2 relaxation time of the MRI signal returned from blood is proportional to its oxygen saturation. The T2 of blood can be measured using an approach called T2 mapping. A series of images of the blood vessels are obtained with different intervals between the excitation of the tissues and the measurements of the returning signal, as shown in Fig. 7.3 . With increasing intervals between excitation and echo, the signal returned from the blood decreases in an exponential manner with time constant T2. By plotting the signal intensity against the time interval, or T2 preparation time, the T2 curve and its time constant T2 can be obtained. The use of adequate spatial resolution and accurate prescription of the imaging plane in the short axis of the vessel are also critical to avoid contamination of the signal being returned by blood with signal from the tissues surrounding the vessel of interest. Fig. 7.4 shows an example of a T2 map made in a normal late-gestation human fetus showing recommended imaging planes for the umbilical vein and descending aorta. The signal is higher in the umbilical vein than the descending aorta, reflecting the higher oxygen saturation in the blood returning from the placenta compared with the blood being supplied to the placenta. In a late-gestation fetus the larger vessels can be imaged using an in-plane resolution of 1.5 × 1.5 mm and a slice thickness of 8 mm, providing ample signal-to-noise ratio. For smaller and more tortuous vessels, higher resolution is ideal, although this may compromise signal-to-noise ratio. However, we have demonstrated the reproducibility of MR oximetry based on T2 mapping in human fetal vessels with a diameter of 5 mm or more. In fetal sheep, in which movement artifacts can be overcome using general anesthesia, we have demonstrated a high degree of correlation between vessel T2 and oxygen saturation measured using conventional blood gas analysis of samples collected using intravascular catheters, as shown in Fig. 7.5 .



To enhance the accuracy of human fetal MR oximetry, the magnetic properties of human fetal blood have been characterized at 1.5 and 3 T using in vitro preparations of umbilical cord blood obtained from elective caesarian sections and prepared through a range of oxygen saturations through graded exposure to nitrogen gas. The T2 of human fetal blood is slightly longer than adult blood. Importantly the relationship between T2 and oxygen saturation is dependent on hematocrit. This is analogous to clinical cyanosis, which is much more obvious in the setting of polycythemia because of the greater amount of deoxyhemoglobin in the blood for the same degree of desaturation. The effect of changes in hematocrit on the relationship between T2 and oxygen saturation is shown in Fig. 7.6 . Thus, to perform accurate oximetry based on T2 mapping, it is necessary to quantify hematocrit. The quantification of hematocrit also allows for an accurate measurement of the oxygen content of blood. To measure hematocrit, the relationship between the T1 recovery of blood and its hematocrit can be utilized. T1 is similar to T2 in that it represents another fundamental magnetic property, this time describing its longitudinal magnetization recovery. The T1 of blood can be measured in a similar fashion to the way we measure T2, by making a series of images with different intervals between and inversion of the magnetization of the blood and the quantification of its signal. A T1 recovery curve for blood is shown in Fig. 7.7 . However, while the T2 of blood is mainly dependent on oxygen saturation but also influenced by hematocrit, the T1 of blood is mainly dependent on hematocrit but also influenced by its saturation. The effect of variation in oxygen saturation on the relationship between T1 and hematocrit are shown in Fig. 7.8 . Using a cubic polynomial solution, it is possible to calculate both oxygen saturation and hematocrit of a blood sample from a combination of its T1 and T2. This is illustrated by the graph in Fig. 7.9 , where the semicircular line represents all of the possible combinations of oxygen saturation and hematocrit for a given R2, where R2 is the T2 relaxation rate or 1/T2. The vertical line represents all the possible combinations of oxygen saturation and hematocrit for a given R1, where R1 is the T1 relaxation rate or 1/T1. The point at which the lines intersect is the only possible solution for hematocrit and oxygen saturation for this particular combination of R1 and R2. Therefore this approach yields blood oxygen saturation, hematocrit, and oxygen content (ignoring oxygen dissolved in plasma), where:
Oxygen content=hemoglobin concentration×oxygen saturation×1.36




The accuracy of the approach has been proven in vitro, as demonstrated by the comparison with conventional blood gas analysis in Fig. 7.10 .

Fetal Hemodynamic Assessment
In a similar approach to the use of the Fick equation for measuring cardiac output based on oxygen consumption and arterial and venous blood oxygen content, the combination of vessel flow measured by phase contrast MRI and vessel oxygen content by MR oximetry can be used to calculate fetal oxygen delivery, oxygen consumption, and oxygen extraction fraction. If we ignore the contribution of oxygen dissolved in plasma (which is negligible in the fetus) then fetal oxygen delivery is the product of umbilical vein oxygen content and umbilical vein flow, while fetal oxygen consumption is given by the difference between umbilical vein and artery oxygen content by umbilical flow. Fetal oxygen extraction is the ratio of oxygen consumption to oxygen delivery. A similar approach can be applied to individual organs, in particular we have been interested to estimate fetal cerebral oxygen delivery and consumption. Unfortunately, the carotid arteries and jugular veins are too small for oximetry and flow measurements in the fetus using our current approach. However, the flow in the SVC is likely to consist primarily of cerebral venous return, with a small and relatively constant contribution from the upper limbs and other parts of the head and neck. Therefore we have approximated fetal cerebral oxygen delivery as the product of SVC flow, aortic arch oxygen content, and fetal cerebral oxygen consumption as the difference between SVC and aortic arch oxygen content by SVC flow.
Normal Late-Gestation Human Fetal Circulation by Magnetic Resonance Imaging
Table 7.1 details reference ranges for the distribution of blood flow across the normal late-gestation human fetal circulation by cine phase contrast MRI with metric optimized gating. These MRI findings are remarkably close to the estimates provided by Rudolph, with the exception of umbilical flow, which was lower than predicted when measured by MRI. However, the umbilical flows we observed by MRI are in keeping with some of the reported ultrasound measurements. In keeping with Rudolph’s observations, we found cerebral blood flow and pulmonary blood flow to be higher in the human than the sheep, while umbilical flow is lower. Rudolph has suggested that the lower umbilical flow seen in the human reflects the higher hematocrit in the human, which provides similar oxygen delivery despite the lower flow. In Tables 7.2 and 7.3 MRI measurements of the oxygen saturations in some of the major human fetal vessels have been provided, as well as fetal oxygen delivery and consumption. Again, these findings are very similar to the fetal sheep, indicating that the same streaming mechanisms are operating to preferentially oxygenate the most metabolically active organs: the brain and the heart. A diagram showing both oxygen saturations and flow distribution in a smaller group of normal late-gestation human fetal subjects is shown in Fig. 7.11 .
CVO | MPA | AAo | SVC | DA | PBF | DAo | UV | FO | |
---|---|---|---|---|---|---|---|---|---|
Mean flow (mL/min/kg) | 477 | 274 | 189 | 146 | 189 | 82 | 255 | 136 | 127 |
SD | 79 | 64 | 35 | 44 | 43 | 52 | 56 | 33 | 56 |
95% CI | 378–664) | 177–435 | 130–255 | 83–259 | 120–279 | 14–190 | 172–385 | 97–202 | 34–226 |
Mean flow (% CVO 2 ) | 57 | 40 | 31 | 40 | 17 | 54 | 29 | 28 | |
SD | 7 | 7 | 9 | 9 | 9 | 10 | 8 | 13 | |
95% CI | 42–67 | 30–55 | 18–50 | 25–57 | 3–29 | 37–75 | 20–46 | 7–54 | |
Modeled mean flow (% CVO 2 ) | 57 | 40 | 29 | 41 | 16 | 52 | 29 | 27 |
Vessel | UV | AAo | MPA | SVC | DAo |
---|---|---|---|---|---|
MRI (mean ± SD) | 84 ± 10 | 69 ± 13 | 55 ± 12 | 38 ± 11 | 56 ± 12 |
Lamb reference | 80 | 65 | 55 | 40 | 60 |
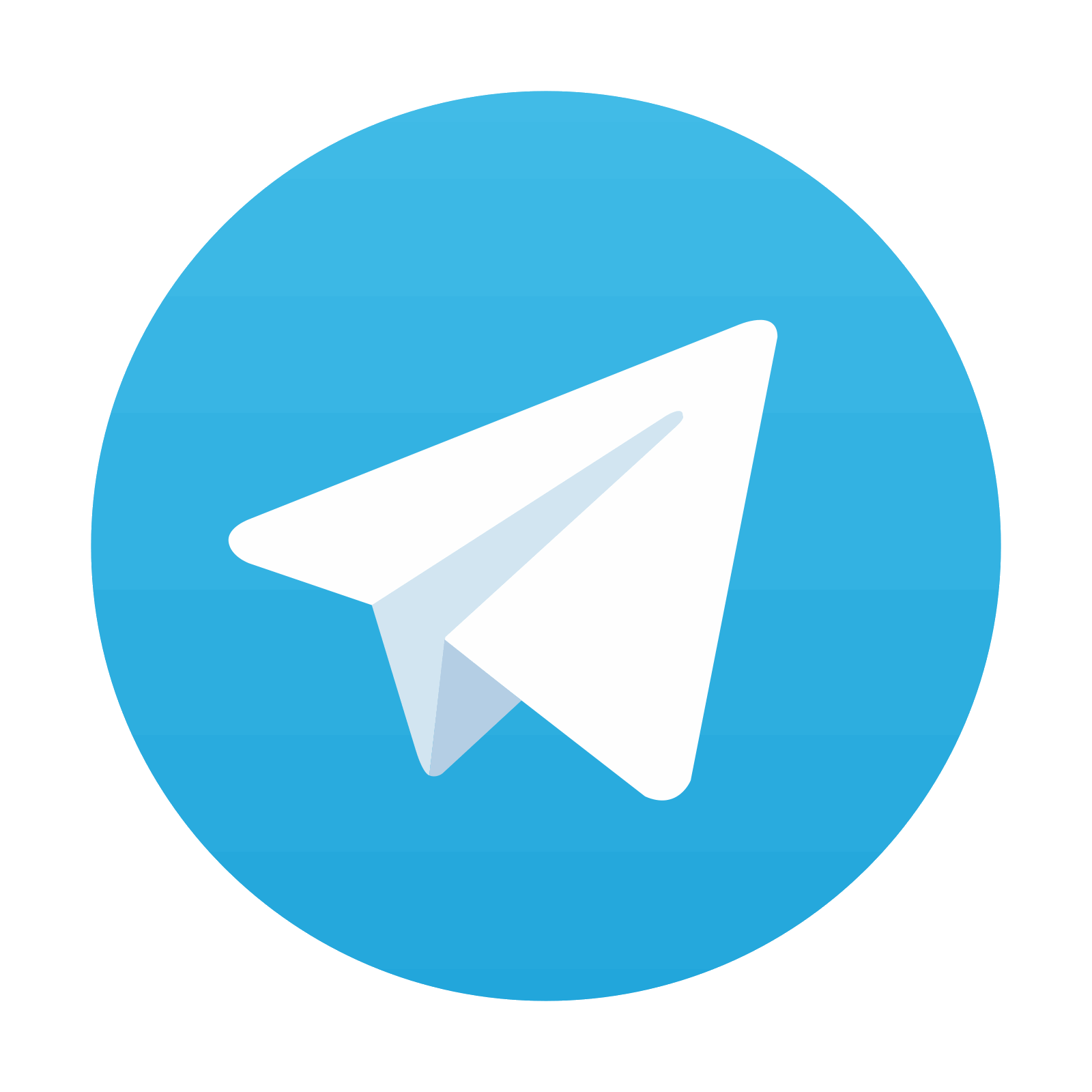
Stay updated, free articles. Join our Telegram channel
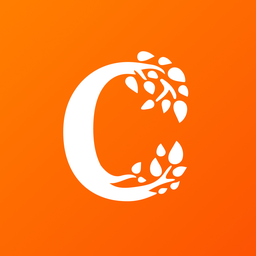
Full access? Get Clinical Tree
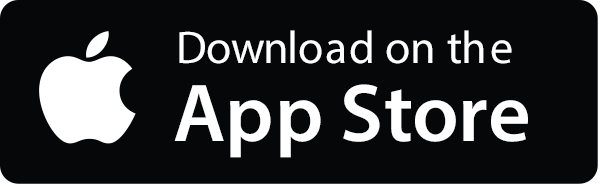
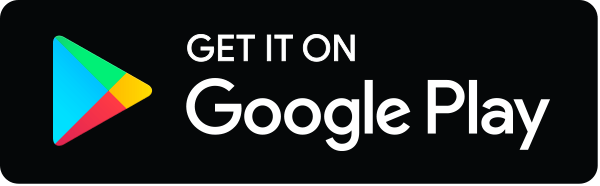
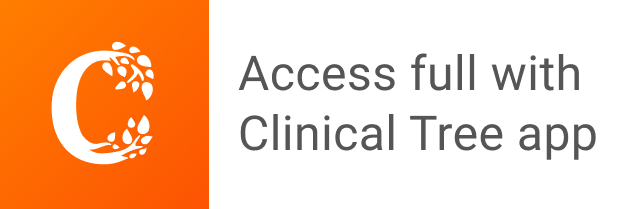