Magnetic Resonance Angiography Techniques
David Saloner
Magnetic resonance (MR) imaging is well suited as a modality for evaluating vascular disease. The principal strengths of MR are that it can provide coverage of an extended vascular territory with information at all points in three-dimensional (3D) space while remaining noninvasive or, as in the case of venous-injected contrast-enhanced imaging, only minimally invasive. The 3D data sets obtained permit display of the vessels in various formats, including contiguous slices that can be oriented in any obliquity or using a projection format. While the major effort in MR angiography (MRA) has been directed at obtaining images of the vascular lumen, there is substantial interest in delineating the disease process itself by imaging the vessel wall. Obtaining data on the dynamics of blood flow, similar to that obtained in catheter-injected x-ray angiography, is also of increasing interest.
As in other MR methods, MRA is least successful when there is substantial gross motion that occurs during the acquisition of the data. This can arise from poor patient compliance or because of physiologic motion, such as cardiac motion while imaging the coronaries or breathing motion for the visceral vessels. Also, blood flow itself can degrade the quality of the depiction of the vascular lumen when that flow is either extremely low, extremely high, or disordered.
Techniques for obtaining high-quality MR angiograms have evolved with improvements in MR instrumentation. As new performance capabilities become available, new techniques become feasible, and this trend can be expected to continue. In this chapter, a number of different methods for obtaining MR angiograms will be discussed. Methods for displaying the data will also be presented.
FLOW DYNAMICS
The signal intensity that is measured in MRA studies of flowing blood depends on blood flow velocities (both magnitude and direction) and the variation of those quantities (e.g., from cardiac pulsatility) over the time that data are acquired. The design and implementation of imaging strategies and the choice of imaging parameters therefore require careful consideration of the anticipated hemodynamics in the vessels, in both the normal and the diseased conditions. Setting aside the problem of gross motion for the moment, MRA methods provide accurate images of the lumen when the image resolution is sufficiently high, to provide several pixels across the diameter of the lumen, where flow is sufficiently high that blood traveling through the imaging volume receives only a small number of radiofrequency (RF) excitations, and where the flow patterns remain regular and reproducible throughout the cardiac cycle. Deviations from these flow conditions can occur in both healthy and diseased vessels (1,2 and 3).
Variations in flow conditions can result from large differences in systolic and diastolic flows. This can be particularly pronounced; for example, in the arteries of the lower extremities where a pronounced interval of rapid antegrade flow is followed by a short, smaller retrograde component, which is, in turn, followed by an extended diastolic interval where the flow velocities are extremely low. In vivo flow patterns are also profoundly impacted by variations in geometry. Even in healthy individuals, blood vessels are curved with numerous branches and bifurcations. These geometric variations can substantially impact the velocity fields resulting in features, such as flattened profiles across the vascular lumen at the entrance to branch vessels or helical flow patterns in curved vessels, such as the aortic arch. However, in the absence of disease, flow is generally laminar and reproducible from cycle to cycle. In regions of atherosclerosis the vascular wall can be irregular, with a residual lumen that is highly asymmetric and that has rapid changes in diameter. These geometric variations can create regions of disturbed flow, such as velocity jets emanating from a tight stenosis accompanied by the shedding of flow vortices representing turbulent flow that varies from one cycle to the next.
PRIMARY MAGNETIC RESONANCE ANGIOGRAPHY METHODS
All MR angiographic techniques aim to create high contrast between spins that are moving and those that are stationary (4,5,6,7,8 and 9). MR imaging methods are capable of measuring both the magnitude of the transverse magnetization and the orientation of that magnetization in space (the phase). Methods have therefore been devised that are designed to create large differences in either the magnitude or the phase of the magnetization between spins that are stationary and spins that are moving (5,7). MR sequences that rely on blood flow to transport fully magnetized blood into the imaging volume and thereby create a substantial difference between the magnetization of flowing and stationary spins are generally referred to as time-of-flight (TOF) methods, which display the magnitude of the transverse magnetization (4). Sequences that rely on the presence of contrast agents injected into the bloodstream to enhance vascular signal are referred to as contrast-enhanced MRA (CE-MRA), and they create images that display the magnitude of the transverse magnetization (10). Images that display the phase of the magnetization are referred to as phase contrast (PC) images (11). These methods rely on the motion of spins, with respect to the imaging gradients for vessel-to-stationary tissue contrast.
FLOW COMPENSATION
Accurate spatial localization, providing images with a high signal-to-noise ratio (SNR), is accomplished by ensuring that in the center of the readout interval, an echo is formed where the orientation of the transverse magnetization of all excited material is the same (rephased). In general, when imaging gradients are applied, spins will accumulate a phase shift. Conventional imaging gradients that are designed to generate a signal echo from stationary material do not account for the motion of flowing spins and, at the center of the echo, moving spins accumulate a phase that depends on their motion between the RF excitation and the time the echo is collected. In a voxel containing blood spins moving with a spread of velocities, various phases occur. The mean magnetization in the voxel is the sum of the individual vectors, and because of the spread of phases, a drop in signal strength, referred to as intravoxel phase dispersion, takes place. For many MR angiographic sequences it is important to implement gradient waveforms such that the magnetization of moving blood is also rephased at the center of the echo (12,13). These are referred to as motion compensation gradients (14). Whereas conventional gradient echo (GRE) sequences use gradient waveforms consisting of two lobes of gradient field strength applied with opposite polarity, flow compensation gradients require additional lobes of applied gradients. In principle, flow compensation could be pursued to include all orders of motion (15). In practice, however, the added gradient lobes needed for higher order motion compensation require additional time to play out, thus lengthening the echo time. If motion compensation is used, the most effective method for improving signal retention from moving spins, even in disturbed flow that contains highorder motion terms, is to rephase constant velocity terms only (16,17,18 and 19).
Most MRA sequences incorporate velocity compensation gradients along the directions of the slice selection and
frequency encoding gradients. The phase encoding direction is not compensated; the short duration of the phase encoding gradients minimizes the effects of motion during the application of those gradients.
frequency encoding gradients. The phase encoding direction is not compensated; the short duration of the phase encoding gradients minimizes the effects of motion during the application of those gradients.
TIME-OF-FLIGHT METHODS
The contrast obtained in an MRA study is closely related to the strength of the longitudinal magnetization in flowing blood relative to that in stationary tissue (6,20,21). In a GRE sequence (flip angle < 90 degrees), the strength of the longitudinal magnetization of spins subjected to a series of RF pulses decreases with each excitation. The longitudinal magnetization of spins will continue to decrease with increasing number of RF excitations received, a process referred to as saturation, until it reaches a steady-state value that is determined by the flip angle, the repetition time (TR), and the T1 relaxation time for that tissue. Stationary material remains in the imaging volume throughout data acquisition and, therefore, the magnetization strength of stationary spins decreases to the steady-state value. In blood vessels, the longitudinal magnetization of spins at any given location depends on how many RF excitations those spins have received between entering the imaging volume and reaching that location. Fast moving blood may receive only a few RF pulses, thus retaining substantial magnetization strength. Slowly moving spins may receive a large number of pulses, and for those spins, their longitudinal magnetization, such as stationary spins, may also drop to the steady-state value. In magnitude images, spins that have received many RF pulses and are strongly saturated will appear dark, whereas spins that retain substantial magnetization strength will appear bright.
TWO-DIMENSIONAL TIME-OF-FLIGHT METHODS
The technique of engendering a strong contrast between the longitudinal magnetization of flowing and stationary spins and then using flow-compensated gradients to correctly encode the transverse magnetization has been used to good effect in thin slice strategies, also called sequential twodimensional time-of-flight (2D TOF) (22,23). In the thin slice strategy, a single slice perpendicular to the blood vessel is acquired using a flow-compensated gradient sequence (Fig. 2.1). By making the slice very thin, it is ensured that the slice will be replenished with relaxed blood and that it does not become saturated, even if moderately large flip angles are applied. Each single slice acquisition requires of the order of 8 seconds. The sequence can be repeated multiple times, each time shifting the position of the slice to permit the acquisition of a large set of consecutive slices in a reasonable imaging time. Note that unlike standard multislice imaging in which the images are collected simultaneously, images are collected sequentially so that blood is not saturated by one slice before entering another. High signal contrast is attained between blood vessels and the stationary surround. This procedure provides a full 3D data set, and the single slices can then be reformatted by stacking them and calculating the desired plane of projection.
![]() Figure 2.1. A 2D GRE sequence slice through the neck. Arteries and veins are shown with high contrast relative to stationary tissue (TR/TE/flip angle = 35/9/30 degrees). |
Shortcomings of Two-dimensional Time-of-flight
Sequential 2D TOF techniques provide strong inflow enhancement when the slices are perpendicular to the vessels. When vessels run in the same plane as the slice, or reenter the slice, the blood becomes saturated, and contrast is progressively lost. This effect is more pronounced when the slices are thick. In addition, in examinations like an axial carotid artery study, a superior presaturation slab is applied, which is close to and moves along with the current slice. This removes venous structures. Vascular loops are sometimes found, especially in the cervical vertebral arteries of older patients. If the loop is large enough, inferiorly moving arterial blood may also be presaturated and yields no signal. For this reason, the method is most effective when the vessel runs in a straight course.
Slices collected perpendicular to the vessel result in projected images in which the plane of viewing, that is, along the length of the vessel, corresponds to the axis with poorest resolution. Resolution will improve, if thin slices are acquired, but this may not be practical because the total imaging time depends on the number of slices collected. In addition, thinner slices have a lower SNR and require longer echo times.
In many cases, particularly in the abdomen, reduced resolution in the projection view can be successfully addressed by acquiring slices in the same plane as the desired projection.
In-plane saturation of the inferior vena cava (IVC) while acquiring coronal slices may be reduced by the use of small flip angles at the expense of contrast.
In-plane saturation of the inferior vena cava (IVC) while acquiring coronal slices may be reduced by the use of small flip angles at the expense of contrast.
THREE-DIMENSIONAL TIME-OF-FLIGHT METHODS
The major shortcoming of the thin slice strategy is the limited resolution that can currently be achieved in the slice selection direction. In 2D techniques using flow compensation gradients, slice thicknesses of 2 mm or greater are typically used. The use of 3D techniques permits the acquisition of a full 3D data set with isotropic voxels of less than 1 mm3 (4,7,24). High-resolution voxels are critical for the visualization of small branch vessels, which might, otherwise, be obscured by partial voluming. They also provide a clear depiction of tortuous vessels with equal fidelity in each spatial dimension.
Three-dimensional methods further permit the acquisition of data sets with echo times that are shorter than those achieved with 2D methods. In acquiring data from a 3D volume, the excitation volume in the “slice select” direction is chosen to be a slab that is of the order of 40 mm thick. Compared to 2D methods, an additional cycle of phase encoding is imposed to permit the data to be reduced to a set of partitions. The use of 3D acquisitions requires substantially greater acquisition times than do 2D methods but provides advantages in increased SNR. In 3D acquisition, blood flowing through the excitation volume receives more excitation pulses than in the case for 2D imaging. To avoid excessive saturation effects, the flip angle must be reduced (<30 degrees). Like other MRI methods, TOF MRA benefits from the increased SNR that is available at high field. This permits the acquisition of images with reduced voxel sizes (Fig. 2.2).
PRESATURATION
In many cases, evaluating an arterial structure is confusing when it is obscured by an overlying vein and vice versa. To eliminate the signal from these vessels, a presaturation band with a flip angle >90 degrees can be placed adjacent to the imaging slice or volume so that the blood is saturated before it enters the slice (Fig. 2.3) (25,26). For example, to remove IVC signal from a renal artery origin study, one could place a transverse presaturation band across the abdomen inferior to the kidneys. Despite their usefulness, the application of presaturation pulses needs careful attention because their existence in a given acquisition is often not directly noted in the resultant images, if they are placed outside the imaging volume. Care must be taken to ensure that the vessel of interest is not similarly presaturated.
PARAMETER CHOICES
In generating an MR angiogram, parameter choices are often made that optimize one feature of the angiogram at the expense of others. In choosing optimum pulse sequence parameters, one needs to take into account the expected flow velocity, the thickness of the imaging volume that must
be traversed, and the acceptable level of contrast between flowing and stationary material.
be traversed, and the acceptable level of contrast between flowing and stationary material.
REPETITION TIME
The selection of the most suitable repetition time is a compromise. The shorter the TR the more saturated the signal from stationary material. However, as TR decreases, blood will receive more RF excitations in traversing the same distance than it would with a larger TR. Figure 2.4 illustrates this, which is a transverse 3D acquisition through the carotid bifurcation from a normal volunteer. The most suitable TR will depend on the rate at which blood transits a slice and will, therefore, vary from vessel to vessel and, for a given vessel, be somewhat patient dependent. One should also
note that an increased TR will dictate a larger total study time and hence, an increased possibility of patient motions, which can substantially degrade image quality.
note that an increased TR will dictate a larger total study time and hence, an increased possibility of patient motions, which can substantially degrade image quality.
FLIP ANGLE
The choice of flip angle, α, is similarly a compromise with increasing α more heavily suppressing stationary signal but more rapidly saturating signal from blood that receives multiple excitations (Fig. 2.5). Again, there is an interaction between the choice of this variable and the flow dynamics. For steady flow, the distribution of magnetization strength across the excited region will reflect the flip angle, and the variation will be larger with increasing flip angle.
As vessels pass through a volume of excitation, blood in the more distal portions becomes increasingly saturated. This
tendency can be counteracted by designing an RF excitation pulse, a TONE pulse, with a flip angle that is relatively small on the proximal side of the slab but that becomes progressively larger across the slab. Spins entering the slab retain their signal strength for a greater distance.
tendency can be counteracted by designing an RF excitation pulse, a TONE pulse, with a flip angle that is relatively small on the proximal side of the slab but that becomes progressively larger across the slab. Spins entering the slab retain their signal strength for a greater distance.
TOF MRA CONSIDERATIONS AND STRATEGIES
Although significant progress has been made using conventional TOF methods, a series of innovative strategies have been pursued to further improve MRA. Specialized sequences have been proposed to reduce the well-known loss of signal noted at sites of disturbed flow. Other approaches have been pursued to enhance the contrast between flowing spins and stationary spins either by more effectively suppressing the signal from stationary spins or by retaining flow signal in the more distal portions of the vessels (as in the TONE approach noted above).
FLOW INDEPENDENT MAGNETIC RESONANCE ANGIOGRAPHY
Conventional MR imaging methods exploit differences in relaxation properties to obtain images with high contrast between different tissue types. It is similarly possible to create images with suitable timing values that exploit the specific T1 and T2 properties of flowing blood and chemical shift differences in surrounding tissue to provide good contrast between blood in the vascular lumen and the surrounding tissue. This type of approach obviates the need for contrast injection and is insensitive to many of the conditions that must be met by MRA methods that are dependent on specific flow properties of intraluminal blood. They do, however, require the careful implementation of a number of advanced methods, such as specialized water-fat suppression methods, and face the challenge of separating arterial from venous signal, which is more easily accomplished at higher field strengths.
DISORDERED BLOOD FLOW
Laminar flow becomes unstable at high flow velocities and breaks down into turbulent flow. In regions where true turbulence exists, such as immediately distal to a critical stenosis, the flow patterns are complicated because some regions will have laminar flow, other regions will have turbulent flow, and intermittent regions exist in which the flow randomly fluctuates between these two conditions. In this case, the different phase encoding steps needed to build up the MRA image will be collected with different signal distribution. These fluctuations will result in inconsistent data and image degradation (27,28). In addition, disturbed flow implies that between RF excitation and signal readout, protons will move through complicated trajectories described not only by constant velocity terms but high-order motion terms that are not rephased by constant velocity compensation. At the time of readout there will be voxels that contain protons with a range of motion histories and a corresponding range of accumulated phases. Such voxels will have a reduced net transverse magnetization strength and will appear in the MR image with reduced signal intensity. The artifactual loss of signal associated with disturbed flow can mimic the appearance of vessel stenosis in vessels of normal caliber and can lead to the overestimation of the degree and extent of stenosis in diseased vessels.
SHORT ECHO SEQUENCES
In general, the effects of disturbed flow that result in intravoxel phase dispersion, can be reduced by shortening the duration of the encoding gradients (17,19,29,30,31 and 32). The extent to which this can be pursued is limited by the rate at which the magnetic field gradients can be altered (the slew rate) and the maximum achievable gradient strength. Further, acquiring data with a strong frequency encoding gradient results in a reduced SNR. Nevertheless, great progress in MRA quality has been achieved with the development of higher performance gradients.
An important consideration in selecting the echo time of TOF MRA sequences is the dependence of the background signal on the echo time. In voxels where there are significant contributions from protons attached to fat and protons attached to water, the signal strength depends on the phase relationship between these two components. The phase relationship is determined by the slight difference in precessional frequencies of protons in the two different environments. At 1.5 tesla (T), this translates to the fat and water signal being out of phase at odd multiples of 2.3 milliseconds and in phase at even multiples of 2.3 milliseconds. As such, images acquired with an echo time of 7 milliseconds will have more effective background suppression than will images acquired at 5 milliseconds (33). Nevertheless, the benefits of short echo time MRA are still apparent in regions where there is little fat contribution, such as the brain.
Reduced echo time sequences can also be achieved by relaxing the requirement that the full echo be placed symmetrically in the data acquisition window. Conventional MRA sequences sacrifice a fraction of the echo and place the echo closer to the excitation pulse to reduce the echo time. The slight loss in resolution that results by dropping the highorder frequency components is compensated by the reduced sensitivity to flow disturbance (34,35). This strategy can be further pursued by using partial Fourier techniques where highly asymmetric echoes are acquired. In this case, the loss in resolution is unacceptable, and additional postprocessing steps must be taken, using the inherent symmetry of the Fourier data to reconstruct the missing information.
SEQUENTIAL THREE-DIMENSIONAL METHODS
Distal saturation of blood flow that occurs in 3D time-off light sequences can also be reduced by reducing the thickness of the excitation slab and by using multiple 3D volumes to cover the region of interest. This method has been termed multiple overlapping thin slab acquisition or MOTSA (36). In this approach, the 3D slabs are placed orthogonal to the principal direction of flow (e.g., axial slabs for studies of the extracranial carotids). To avoid bands of signal loss where the RF profiles trail off at the slab edges, substantial overlap of consecutive slabs is provided. This comes with a penalty
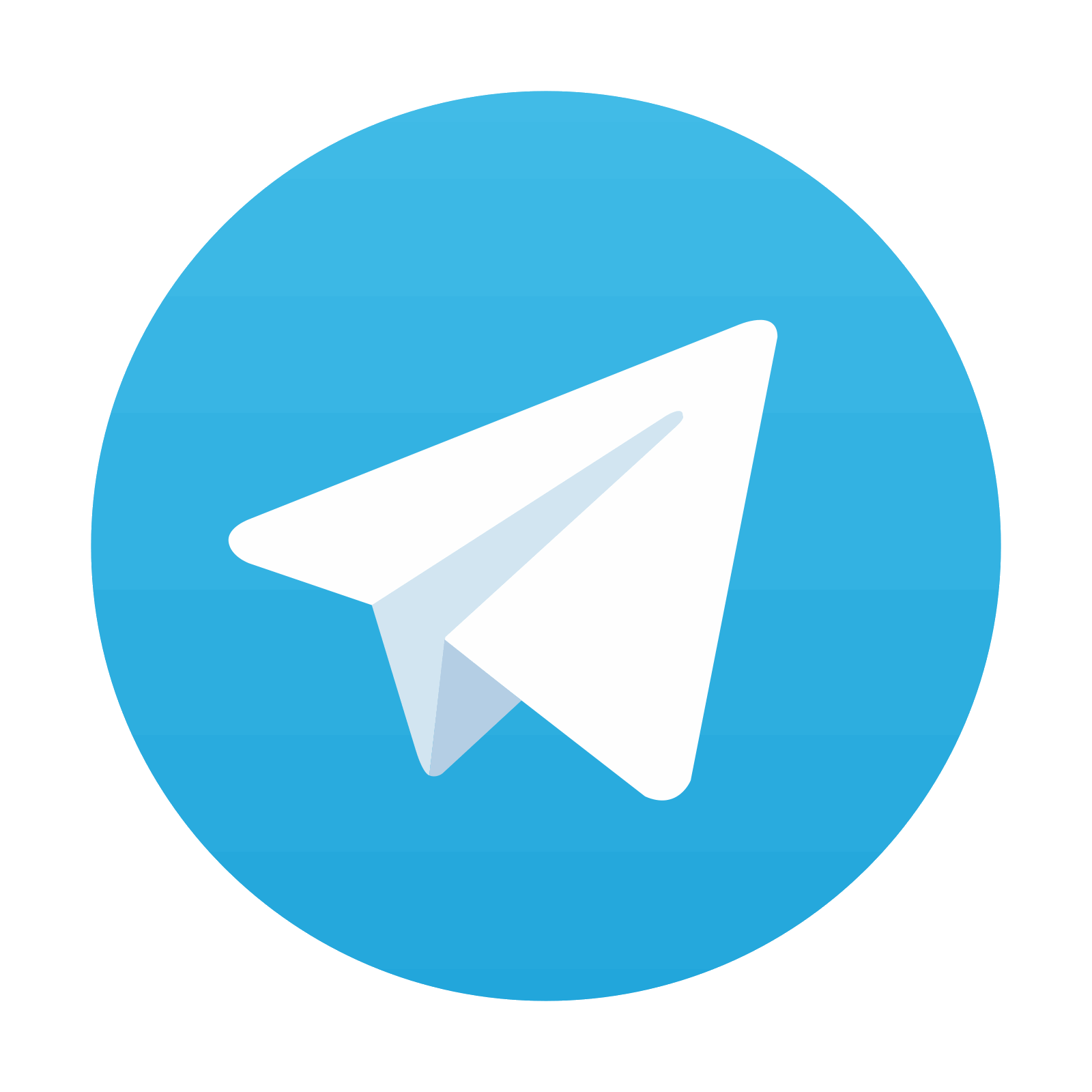
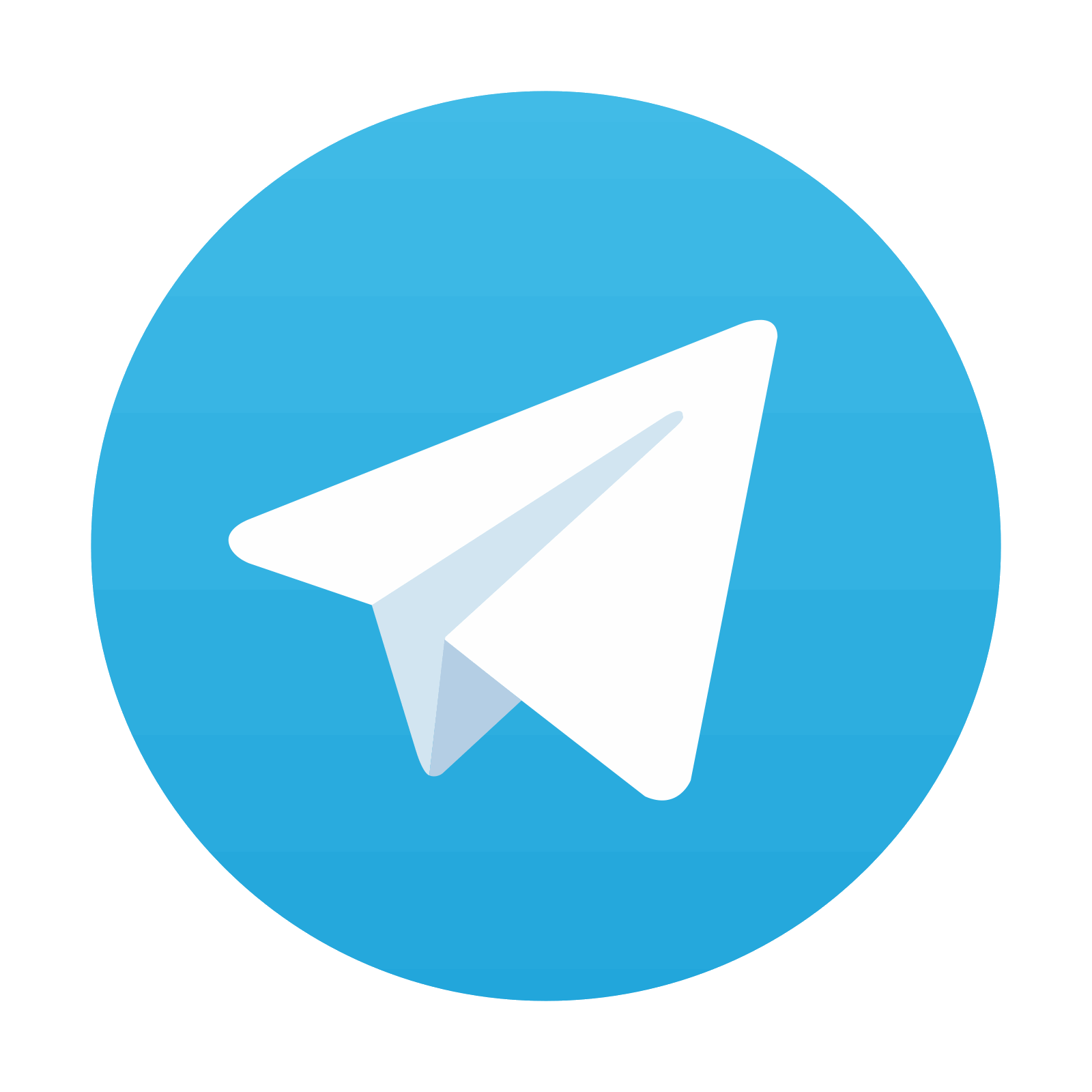
Stay updated, free articles. Join our Telegram channel
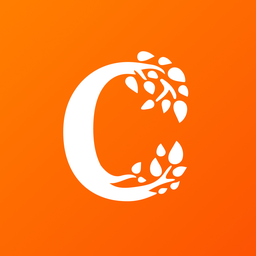
Full access? Get Clinical Tree
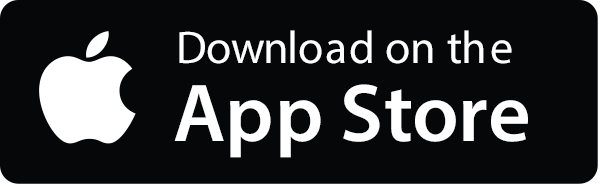
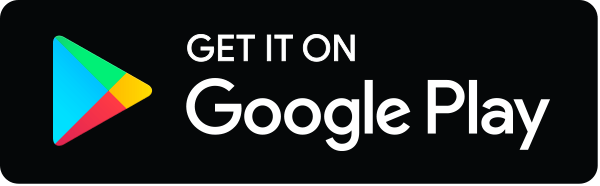
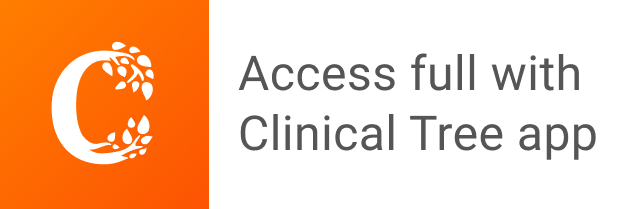