Magnetic Resonance Angiography in the Diagnosis of Vascular Disease
Paaladinesh Thavendiranathan
Georgeta Mihai
Magnetic resonance angiography (MRA) is an excellent modality that has become the noninvasive modality of choice for imaging in a number of vascular beds. MRA refers to a set of pulse sequences in magnetic resonance imaging (MRI) that have been adapted for the clinical imaging of the lumen and the walls of vascular structures. These sequences take advantage of and/or obviate specific properties of blood, blood flow, or vascular walls or use gadolinium (Gd)-based contrast agents to enable visualization of vascular structures with high resolution.
FUNDAMENTALS OF MAGNETIC RESONANCE IMAGING
The Physics of Magnetic Resonance Imaging
MRI is based on two properties of the hydrogen nucleus (called either proton or spin) to produce images of biological objects: its magnetic moment (similar to a small bar magnet) and its angular momentum or spin. When a proton is placed under a strong magnetic field B0 (typically 10,000 times greater than the earth’s magnetic field), its magnetic axis aligns itself with B0 and experiences a motion of precession (similar to a top) around the direction of B0. The frequency of the precession, called the Larmor frequency, is proportional to the intensity of the local magnetic field experienced by the proton. Resonance is referred to as the property of a nucleus with an odd number of protons or neutrons to selectively absorb and later release energy specific to the element and its chemical environment. When a short radiofrequency (RF) excitation pulse—B1, with a frequency that matches the Larmor frequency of protons, is applied on an object placed under a strong magnetic field, the resonance phenomenon occurs and its protons synchronize their precession and have their magnetic axis tipped out of alignment with B0. The angle of net magnetization-deflection created after the end of the RF pulse is referred to as the flip angle and is dependent on the strength of the externally applied field B1 and the duration of the RF pulse. A 90 degrees RF pulse will rotate the net magnetization totally from longitudinal plane (z, or B0 plane) to transversal plane (xy). It is the transversal component (Mxy) of the net magnetization that generates the MR signal in the form of an oscillating current induced in a receiver antenna placed perpendicular on the xy plane. After the RF excitation pulse ends, two concurrent events happen: (i) Mxy starts to decay (with a time constant T2 called transversal or spin-spin relaxation) due to the loss of phase coherence among spins (as each spin “feels” a different
magnetic field environment dependent on the neighboring spins), and (ii) the magnetic axes of the protons realign themselves with B0 with a time constant T1 (longitudinal or spin-lattice relaxation time). The oscillating signal produced by the decay in time of Mxy is called free induction decay (FID) and is dependent on the local magnetic environment surrounding each proton. The spin-spin interaction is not the only factor responsible for the loss of phase coherence among spins and the time decay of Mxy. Extrinsic magnetic filed inhomogeneities (magnetic field imperfections and/or susceptibility differences between adjacent tissues) accelerate the MRI signal decay that becomes characterized by a time constant T2*, which is always shorter than T2. However, in spin-echo sequences, the signal loss caused by static, extrinsic magnetic field inhomogenities is corrected by the use of the 180 degrees RF pulse that reverses the phase differences and reestablishes phase coherence allowing “echo formation,” as such the signal decay is only T2 dependent. In gradient-echo imaging, where different polarity gradients instead of the 180 degrees RF pulse are used to form an echo, the T2* FID is observed. The loss of phase coherence among spins occurs more rapidly than the longitudinal magnetization recovery, as such always T1 > T2 > T2*. T1, T2, and spin (proton) density (PD, number of spins per unit tissue area) are fundamental, intrinsic properties of tissue that could be exploited by MRI to differentiate tissues.
magnetic field environment dependent on the neighboring spins), and (ii) the magnetic axes of the protons realign themselves with B0 with a time constant T1 (longitudinal or spin-lattice relaxation time). The oscillating signal produced by the decay in time of Mxy is called free induction decay (FID) and is dependent on the local magnetic environment surrounding each proton. The spin-spin interaction is not the only factor responsible for the loss of phase coherence among spins and the time decay of Mxy. Extrinsic magnetic filed inhomogeneities (magnetic field imperfections and/or susceptibility differences between adjacent tissues) accelerate the MRI signal decay that becomes characterized by a time constant T2*, which is always shorter than T2. However, in spin-echo sequences, the signal loss caused by static, extrinsic magnetic field inhomogenities is corrected by the use of the 180 degrees RF pulse that reverses the phase differences and reestablishes phase coherence allowing “echo formation,” as such the signal decay is only T2 dependent. In gradient-echo imaging, where different polarity gradients instead of the 180 degrees RF pulse are used to form an echo, the T2* FID is observed. The loss of phase coherence among spins occurs more rapidly than the longitudinal magnetization recovery, as such always T1 > T2 > T2*. T1, T2, and spin (proton) density (PD, number of spins per unit tissue area) are fundamental, intrinsic properties of tissue that could be exploited by MRI to differentiate tissues.
Spatial Localization and K-Space
Field Gradients
Field gradients (linear, controlled variations in the B0 strength) make MRI possible by allowing the MR signal to be localized in space. These linear changes in B0 translate into linear, location-dependent changes in the Larmor frequency of the spins. By precisely controlling the strength of the magnetic field in space and time, the frequency and phase of spin precession also become a function of location and time. An image can be formed as the MR signals coming from different locations within the body can be distinguished from one another. The gradients are applied in three planes as slice select, phase encode, and frequency (readout) gradients. The exact timing, order, polarity, repetition frequency of the RF pulses, and applied gradient fields are specific to each pulse sequence (used to emphasize different types of contrast information in the tissues of the object). The specific succession of RF pulse, gradients, and echo acquisitions has to be repeated numerous times (number of repetitions = acquired matrix size). The time between successive RF pulses is referred to as repetition time (TR), while the time between RF pulse and echo is referred to as echo time (TE).
k-Space
During a pulse sequence, after each echo acquisition, the resulting echoes are digitized and stored in a data acquisition matrix using Fourier transformation methods. This data matrix is referred to as κ-space. Tissue contrast is principally determined by the center of κ-space (central phase encoding lines), while the periphery encodes image detail. The order in which κ-space lines are collected can be varied (phase reordering) and strongly influences
tissue contrast and detail. In conventional MRI, the κ-space acquisition is sequential, while in fast MRI techniques for three-dimensional (3D) contrast angiography, centric or elliptic-centric acquisition is often used. The κ-space data set is Fourier transformed to produce the images that are routinely seen in clinical practice.
tissue contrast and detail. In conventional MRI, the κ-space acquisition is sequential, while in fast MRI techniques for three-dimensional (3D) contrast angiography, centric or elliptic-centric acquisition is often used. The κ-space data set is Fourier transformed to produce the images that are routinely seen in clinical practice.
General Magnetic Resonance Imaging Pulse Sequences and Fast Vascular Imaging
Pulse sequences that are routinely used in MRI can be classified into two main categories: gradient-recalled echo (GRE) sequences and spin-echo (SE) sequences. In GRE sequences, following the initial RF pulse, opposite polarity, same area gradients are used to dephase and rephase the protons in the transverse plane in order to obtain an echo and the MR signal. These sequences generally use an RF pulse with a flip angle less than 90 degrees. This is advantageous in fast imaging approaches such as contrast angiography as there is always residual magnetization left after application of the RF pulse, obviating the need to wait long times to allow sufficient longitudinal recovery. Even though GRE sequences are faster than SE sequences, they often result in lower signal-to-noise ratio (SNR) images due to use of low flip angle and often require T1-shortening contrast agents to enhance SNR. In SE sequences, following a 90 degrees RF pulse, 180 degrees RF pulses are applied to rephase the protons in the xy plane in order to obtain an MR signal. In fast SE techniques, multiple 180 degrees pulses can be applied for each 90 degrees pulse allowing for collection of multiple lines of κ-space during a TR interval. However, generally SE sequences result in slower imaging than GRE. T1, PD, T2* weighting and a contrast based on the ratio of T1/T2 of tissues can be obtained in GRE sequences, while T1-, PD-, and T2-weighted contrast can be obtained with SE sequences. Both GRE and SE sequences are routinely utilized in vascular MRA.
A prerequisite of vascular imaging approaches is the ability to rapidly image the vasculature that often spans large territories. An approach that is commonly used to speed imaging is parallel imaging. Parallel imaging techniques use the spatial information inherent in local phased-array coils to replace time-consuming phase encoding steps and can be subdivided into the domains (κ-space/image-space) in which processing is mainly performed. SMASH (simultaneous acquisition of spatial harmonics) and GRAPPA (for generalized autocalibrating partially parallel acquisition) are techniques that acquire local coil information in Fourier space that is then used to reconstruct the image. In contrast, the processing in SENSE (for sensitivity encoding) is performed in the imaging domain by acquisition of local coil data and application of correction after the Fourier transform.
Magnetic Resonance Angiography Pulse Sequences for Vascular Imaging
MRA pulse sequences can be categorized into (a) flow-dependent versus independent sequences, (b) “black-blood” versus “bright-blood” sequences, or (c) contrast-enhanced versus unenhanced sequences. The most practical classification is to consider these sequences as black-blood or brightblood techniques based on the appearance of the vascular lumen following
application of certain pulse sequences. Thinking of these sequences as contrast-enhanced (CE) versus unenhanced sequences is also important as it has practical implications in imaging patients with contraindications to Gd-based contrast administration.
application of certain pulse sequences. Thinking of these sequences as contrast-enhanced (CE) versus unenhanced sequences is also important as it has practical implications in imaging patients with contraindications to Gd-based contrast administration.
Black-blood Techniques
Black-blood imaging techniques are useful for assessing vessel wall pathologies such as atherosclerosis, aneurysms, intramural hematoma, aortic dissections, and vasculitides. Generally black-blood sequences are fast SE sequences with preparation modules for blood signal suppression. These sequences are mostly two dimensional (2D), allow for depiction of lumen and vascular wall, are able to deliver all contrast types (T1, T2, and PD), and need efficient suppression of the flowing blood. The latest is accomplished either by inflow-outflow saturation bands (flowing blood experiences an extra 90 degrees RF pulse outside the imaging slice) or double inversion recovery (IR) or even triple IR to null the flowing blood. Blackblood techniques are all noncontrast sequences making them useful in patients with contraindications to Gd contrast agents.
Partial Fourier Acquisition Methods
Partial Fourier methods appeal to vascular imaging due to the speed of acquisition. Half-Fourier Acquisition Single-Shot Turbo Spin-Echo (HASTE) imaging is a rapid SE sequence where the entire 2D slice dataset data is acquired in a heart beat (TR = R — R interval) and is accomplished using very short inter-echo spacing, acquisition of slightly more that 50% of the κ-space and mathematically interpolating the remaining κ-space lines. For MRA applications, HASTE sequences are executed with preparatory double inversion pulses with specific inversion times, to selectively null signal from flowing blood. These sequences are typically implemented with ECG gating, with image acquisition during diastolic period to avoid flowrelated artifacts. Due to the partial Fourier acquisition, spatial resolution and SNR are inferior compared to full κ-space acquired fast SE methods. However, the rapidity of image acquisition reduces cardiac and respiratory motion artifacts allowing imaging of large vascular areas within 1 to 2 minutes with the patient breathing freely. Typically, coronal, axial, and sagittal HASTE images are obtained and can be used for diagnostic purposes as well as subsequent planning of contrast-enhanced MRA sequences.
Another partial Fourier approach is an ECG-gated 3D sequence which is triggered for systolic and diastolic acquisitions. This method relies on loss of signal or flow void during systole as a result of fast arterial flow. The diastolic acquisition is characterized by slow flow and high-signal intensity on T2-weighted images. Bright-blood angiography can then be accomplished by simple Fourier subtraction of the images.
Bright-blood Techniques
While dark-blood techniques focus on signal loss due to flow, bright-blood imaging uses flow-related enhancement or Gd-based contrast agents to enhance signal from blood. Bright-blood sequences are generally GRE
sequences. Time-of-flight (TOF) MRA, phase-contrast (PC) imaging, and steady-state free precession (SSFP) imaging with navigator gating are noncontrast techniques, while 3D CE-MRA and time-resolved MRA (TR-MRA) require the use of contrast.
sequences. Time-of-flight (TOF) MRA, phase-contrast (PC) imaging, and steady-state free precession (SSFP) imaging with navigator gating are noncontrast techniques, while 3D CE-MRA and time-resolved MRA (TR-MRA) require the use of contrast.
Balanced Steady-state Free Precession Magnetic Resonance Angiography. Balanced SSFP imaging is a GRE technique where the image contrast is determined by the intrinsic T2/T1 differences between blood and tissue. In this sequence, RF pulses of alternating direction are applied with a TR much shorter than T1, hence maintaining residual Mxy. This residual magnetization is used to increase final signal intensity resulting in bright-blood images with no reliance on flow. The high SNR of this sequence allows it to be well suited for parallel imaging. Balanced SSFP imaging can be used to obtain 2D datasets, cine images, or 3D datasets using navigator-gated free breathing techniques. In the latter application, 3D-MRA is acquired using ECG gating to trigger for diastolic acquisitions, as well as respiratory triggering to obtain 3D datasets in 5 to 10 minutes. These sequences are however susceptible to field inhomogeneities including air-tissue interfaces, metallic implants, and stents. Additionally, these sequences are not specific for veins or arteries, as both structures appear bright in the images.
Time-of-flight Magnetic Resonance Angiography. In TOF imaging, a series of RF pulses is used to “saturate” all protons in an imaging volume and suppress the signal from all stationary tissue within that volume. Blood flowing into the imaging volume brings “unsaturated” protons that have not experienced the prior excitation pulses of the other protons stationary in the imaging plane and produce higher signals. In 2D-TOF, thin slices are excited, whereas in 3D-TOF, thicker volumes are excited. The application of 2D or 3D techniques is dependent on velocity of blood flow. In general, flow that is too slow to cross the entire volume between excitations may partly saturate with parts of it producing no signal. Thinner slices, as used in 2D-TOF, are therefore better suited to image slow-flowing blood. Additional saturation pulses immediately above or below the plane of imaging can be used to selectively suppress signals from either veins or arteries, allowing the respective production of MRA and MR venograms (MRV).
Phase-contrast Imaging. In PC imaging, bipolar magnetic gradient fields perpendicular to the plane of imaging are used to quantify the speed of spatially moving protons. PC method relies on velocity of the moving spins to produce signal, and as such, there is excellent background suppression. A short-duration gradient is applied to a vessel followed by an opposite and equal gradient for the same duration. As a consequence, stationary protons do not gain a net phase. However, for protons moving perpendicular to the plane of imaging, the effect of the first gradient field is not perfectly counteracted by the second gradient field, resulting in a phase offset. The greater the velocity of the moving protons, the greater the resulting phase offset. These phase offsets can then be used to calculate velocity. Given that phase can only take values between -180 and +180 degrees, a net offset in phase of 200 degrees is identical to an offset of -160 degrees.
The intensity of the bipolar gradient fields must thus be adjusted according to the maximum expected velocity (Vmax or VENC, velocity encoding) to maintain all phase offsets within the -180- to +180-degree interval and avoid aliasing.
The intensity of the bipolar gradient fields must thus be adjusted according to the maximum expected velocity (Vmax or VENC, velocity encoding) to maintain all phase offsets within the -180- to +180-degree interval and avoid aliasing.
3D Contrast-enhanced-Magnetic Resonance Angiography. 3D CE-MRA uses a 3D spoiled gradient-echo (SPGR) pulse sequence (rapid imaging) to optimize imaging of the first pass of a Gd-based contrast agent in vessels. The contrast agent shortens the T1 of blood, increasing the natural contrast between the blood and the surrounding tissues. A large enough dose of contrast agent must be administered to lower the T1 of blood below that of the surrounding fat for good signal contrast between vessels and perivascular tissue. A typical dose of Gd contrast is 0.1 to 0.3 mmol/kg and is injected at a rate of at least 2.0 mL/s. Timing of the contrast bolus with respect to imaging is important. A technical trick can be used where the central part of κ-space (reflecting the global contrast in an image) alone is acquired during the peak of concentration of the bolus in the arteries of the targeted anatomy, thus shortening the time of acquisition. High-resolution arterial phase images of the lumen with high contrast delineation may be obtained when combined with parallel imaging techniques.
Time-resolved Magnetic Resonance Angiography. TR-MRA is a technique of assessing the kinetics of contrast enhancement in a chosen vascular bed. It uses fast imaging methods to acquire multiple MRA images with high temporal resolution to demonstrate the dynamics of contrast enhancement during first-pass of the contrast bolus. There is repetitive filling of the center of k-space as the contrast bolus is injected, followed by later filling of the remainder of k-space. TR-MRA techniques when used in conjunction with parallel imaging can effectively reduce temporal resolution to 200 to 300 ms/3D imaging slab. The rapid image acquisition obviates the need for precise arterial contrast timing and provides selective arterial enhancement. However, the downside of performing rapid imaging is the compromised (low) spatial resolution of the images.
Noncontrast Approaches
These approaches may provide either black-blood or bright-blood images, depending on the sequence. These include Sampling Perfection with Application of optimized Contrast using different flip angle Evolution (SPACE) (see below), SSFP (bright-blood approach described above), and 3D ECGgated partial Fourier fast-spin echo approaches and arterial spin labeling (both, typically dark blood). The application of these in various beds is described in Table 4.1. For detailed reviews on noncontrast approaches, the reader is referred to detailed reviews on the topic.
3D-sampling Perfection with Application of Optimized Contrast Using Different Flip Angle Evolution
The SPACE technique is a new 3D dark-blood method used for vascular imaging that belongs to the family of turbo-spin echoes and is still used mainly for investigational purposes. This sequence utilizes variable refocusing flip
angles allowing for longer echo train duration and nonselective refocusing pulses to minimize echo spacing. This significantly improves its time efficiency over conventional 3D fast-spin echo sequences. Additionally, flowing blood is dephased and blood signal is suppressed without the need for double inversion preparation pulses, hence allowing the use of thick 3D slabs and efficient coverage of large vascular territories. To acquire large slabs of vascular structures such as the thoracic and abdominal aorta, respiratory gating is used with the patient breathing freely during acquisition as the acquisition can take 10 to 15 minutes. SPACE can achieve any of the SE contrasts (T1, T2, PD). Other than for vessel wall pathology, SPACE can be used when significant stent susceptibility artifact precludes the use of GRE sequences, as it allows imaging outside of the stent without any signal loss.
angles allowing for longer echo train duration and nonselective refocusing pulses to minimize echo spacing. This significantly improves its time efficiency over conventional 3D fast-spin echo sequences. Additionally, flowing blood is dephased and blood signal is suppressed without the need for double inversion preparation pulses, hence allowing the use of thick 3D slabs and efficient coverage of large vascular territories. To acquire large slabs of vascular structures such as the thoracic and abdominal aorta, respiratory gating is used with the patient breathing freely during acquisition as the acquisition can take 10 to 15 minutes. SPACE can achieve any of the SE contrasts (T1, T2, PD). Other than for vessel wall pathology, SPACE can be used when significant stent susceptibility artifact precludes the use of GRE sequences, as it allows imaging outside of the stent without any signal loss.
TABLE 4.1 PREFERRED NONCONTRAST MRA TECHNIQUES BY LOCATION | ||||||||||||||||||
---|---|---|---|---|---|---|---|---|---|---|---|---|---|---|---|---|---|---|
|
Magnetic Resonance Imaging Approaches for Tissue Characterization
These can be part of MRA protocols and may help with interrogation of pathology in the arterial wall and are helpful in characterization of atherosclerotic plaque and complications such as thrombosis, which may occur as part of diverse arterial and venous disorders. Tissue characterization relies on the intrinsic signal characteristics of pathology such as iron, methemoglobin, or thrombus and can be discerned by their image characteristics on specific pulse sequences. These include T1-weighted SE or GRE sequences with and without IV contrast, T2* images, or T2-weighted SE imaging.
Magnetic Resonance Digital Subtraction Angiography
Image contrast can be improved by digital subtraction of precontrast image data from dynamic, arterial, or venous phase image data. This subtraction is typically performed prior to the Fourier transform by
using a complex subtraction method. The improvement in contrast achieved with DSA may reduce the Gd dose required. However, there must be no change in the patient position between the precontrast and dynamic/post-contrast-enhanced imaging. This requirement for no motion is easily met in the pelvis and legs, which can be sandbagged and strapped down. It is more difficult to achieve in the chest and abdomen, where respiratory, cardiac, and peristaltic motions are more difficult to avoid.
using a complex subtraction method. The improvement in contrast achieved with DSA may reduce the Gd dose required. However, there must be no change in the patient position between the precontrast and dynamic/post-contrast-enhanced imaging. This requirement for no motion is easily met in the pelvis and legs, which can be sandbagged and strapped down. It is more difficult to achieve in the chest and abdomen, where respiratory, cardiac, and peristaltic motions are more difficult to avoid.
CLINICAL APPLICATIONS OF MAGNETIC RESONANCE ANGIOGRAPHY IN VASCULAR DISEASE
Carotid and Verterbral Magnetic Resonance Angiography
Indications
MRA can delineate carotid bifurcation disease, aortic arch branch vessel disease, stenosis and occlusion, atherosclerotic disease, fibromuscular dysplasia, carotid and vertebral artery dissection, vascular neoplasms, and carotid artery aneurysms. A more contemporary application of MRA (using mostly dark-blood techniques) that is likely to become a valuable tool for therapeutic planning in the future is carotid plaque characterization to detect high-risk components within atherosclerotic plaques. This is however still experimental at present.
Approach
3D CE Angiography
3D CE-MRA has revolutionized noninvasive examination of the carotid and aortic arch vessels and has replaced intra-arterial angiography in many institutions. Figure 4.1 illustrates an MRA of the extracranial carotid arteries, revealing a tight stenosis at the origin of the left internal carotid artery. 3D CE-MRA of the carotid is usually obtained in the coronal plane and does not require breath-holding. The acquisition is performed with centric κ-space weighting from the beginning of the scan to avoid jugular venous enhancement. Arterial-venous transit time in the cerebral circulation is rapid, necessitating split-second timing typically executed with a timing bolus.
Flow Measurements. PC imaging can be performed in areas identified to assess hemodynamic significance of stenosis or flow direction using in-plane acquisitions as well as to quantify the severity by using through plane acquisitions.
Tissue Characterization. For vessel wall and plaque imaging, other more standard MRI sequences such as double and triple IR, T1-weighted pre-IV and post-IV contrast, and T2-weighted sequences can also be performed in chosen areas. Many of these however have to be performed precontrast administration.
Typical Protocols
A typical MRA of the extracranial circulation begins with anatomic localizers in the transverse, sagittal, and coronal planes. Dark-blood 3D T1- or T2-weighted SPACE imaging of the carotids can be subsequently performed for plaque characterization (however, this is mainly a research tool at present). This is followed by coronal 3D CE-MRA through the extracranial carotids in a single injection. Inclusion of the arch and the origins of all supra-aortic arteries in the field of view is important. If an area of stenosis is detected, sagittal 2D PC slabs positioned on the right or left carotid can be performed to assess for presence of turbulence. Maximum intensity projections (MIP) reconstructions are typically performed for global assessment of carotid pathology. In cases of subclavian artery stenosis, an axial PC MRA at the level of the proximal neck may be informative to deduce direction of flow in the ipsilateral vertebral artery.
Thoracic Magnetic Resonance Angiography
Noninvasive techniques of computed tomographic angiography (CTA) and MRA have completely replaced diagnostic catheter angiography of the thoracic vessels. The ability to obtain physiologic information and vessel wall information is a particular advantage of thoracic MRA.
Indications
MRA is able to accurately assess pathology of the great vessels in the chest. This includes the thoracic aorta and its branches, the pulmonary arteries and their branches, and the large veins of the chest. Imaging of the coronary arteries and veins is possible by MRI but is not widely used clinically.
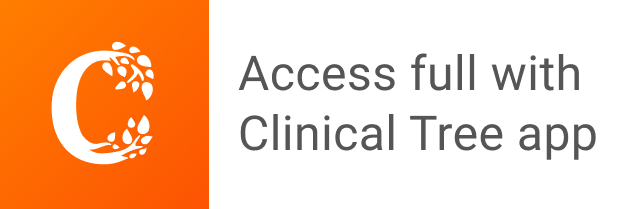