Magnetic Resonance and Computed Tomography Angiography of Peripheral Arteries
Martin N. Wasser
Harrie C.M. van den Bosch
With the increasing average age of the population in the industrialized world, atherosclerotic disease of the peripheral vessels is gaining importance. Although atherosclerotic lesions occur in all vascular territories, the lower limbs are affected in 90% of cases. Presently, atherosclerosis in the lower limbs accounts for 50,000 to 60,000 percutaneous angioplasties, implantation of 110,000 vascular prostheses, and 100,000 amputations annually in the United States (1).
Before the planning of therapeutic actions, detailed information on localization, extent, and severity of the disease is essential. Conventional angiography has long served as the imaging modality of choice in this respect. However, x-ray angiography has definite risks and limitations, including the possibility of a severe contrast medium reaction, even when nonionic agents are used (2). Also, because approximately 70% of patients with severe occlusive peripheral disease have evidence of impairment of renal function (3), contrast-induced renal insufficiency is a matter of concern. Therefore, noninvasive alternatives, such as duplex ultrasound and magnetic resonance (MR) angiography, are increasingly used in the diagnostic workup of patients with peripheral arterial disease.
Contrast-enhanced magnetic resonance angiography (CEMRA) has rapidly emerged as an attractive alternative to conventional angiography. The reason for this rapid acceptance in the “vascular community” is the close resemblance of CE-MRA images to conventional angiography. The contrast agent gadolinium diethylene triamine pentaacetic acid (DTPA) in the dosages used for MRA has long been considered non-nephrotoxic (4). The recent association between high doses of gadolinium-based contrast material and nephrogenic systemic fibrosis (NSF) (5,6), however, has made it imperative to consider nonenhanced alternatives for angiography in patients with moderate to severe renal insufficiency and vascular disease. Fortunately, it appears that the occurrence of NSF is related to the formulation of the gadolinium compound being applied and the contrast agents that have been associated with NSF have been taken of the market.
With the advent of multidetector row or multislice computed tomography (MSCT), another modality has emerged for the noninvasive evaluation of the peripheral vasculature. Although this technique involves the use of radiation and iodinated contrast agents, the spatial resolution that can
be obtained with multislice CT angiography (CTA) is superior to that of MR angiography. CTA has therefore rapidly become a serious competitor for MRA in evaluation of peripheral vascular disease in patients with nonimpaired renal function.
be obtained with multislice CT angiography (CTA) is superior to that of MR angiography. CTA has therefore rapidly become a serious competitor for MRA in evaluation of peripheral vascular disease in patients with nonimpaired renal function.
This chapter will provide an overview of the techniques and indications for CE-MRA and CE-CTA of the peripheral arteries of the lower limbs. Although atherosclerosis is the major cause of peripheral arterial disease, other abnormalities will also be addressed.
MAGNETIC RESONANCE ANGIOGRAPHY OF PERIPHERAL ARTERIES
ENHANCED VERSUS UNENHANCED MRA
The older unenhanced MRA techniques [phase-contrast MRA (PCA) and time-of-flight (TOF) MRA] suffered from artifacts caused by turbulence and in-plane saturation. These techniques were also highly time consuming. An important reason for the long acquisition times in conventional MRA is the requirement of obtaining images in the transverse plane to circumvent in-plane saturation. Due to the triphasic flow pattern in the peripheral vessels with diastolic flow reversal, cardiac triggering has to be performed in conventional MRA in order to prevent erroneous signal loss. This cardiac triggering causes additional lengthening of the study.
In 2000, a meta-analysis was published in which the results of 21 studies on peripheral MRA published in the literature from January 1991 through June 1999 were evaluated (7). The sensitivity for detection of hemodynamically significant stenosis (>50% luminal reduction) ranged from 64% to 100% for two-dimensional (2D) TOF and 92% to 100% for three-dimensional (3D) CE-MRA. Specificity ranged from 68% to 96% for 2D TOF and 91% to 99% for 3D CE-MRA. This indicates that 3D CE-MRA is superior to 2D TOF for the detection of peripheral arterial disease. Especially for imaging of the proximal vessels in the legs 2D TOF appears to be less reliable (sensitivity 83% to 100%, specificity 23% to 98%). In tortuous, elongated iliac arteries, 2D TOF may show falsepositive results due to in-plane saturation or saturation of arterial signal by the venous presaturation slab. Short segments of signal void may be caused by either a short occlusion or a severe stenosis. Also, retrograde flow may not be recognized due to the venous presaturation slab.
Also in the femoropopliteal and infrapopliteal regions CE-MRA performed better than 2D TOF. Sensitivity ranged between 88% and 92% and between 94% and 100% for 2D TOF and CE-MRA, respectively (specificity 82% to 98% vs. 97% to 99%). With respect to the infrapopliteal vessels sensitivity/specificity were 89% to 98%/91% to 95% versus 91% to 94%/100% for 2D TOF and CE-MRA, respectively.
No figures exist for the accuracy of MRA for arterial disease in the upper limbs. Although conventional MRA and CE-MRA have not been compared, there is no reason to doubt that CE-MRA is also the best technique to evaluate arteries in this territory. Similar to arteries in the lower extremities, arteries in the arms show a triphasic, high-resistance flow pattern with identical artifacts on conventional MRA.
The discovery of association between Gd-compounds and NSF has led to a renewed interest in nonenhanced MRA. Improvements in MR hardware and software, including the widespread availability of parallel imaging have helped to reduce acquisition times and have made some methods clinically practical. Nonenhanced MR angiography may play a useful role as a supplement to gadolinium-enhanced MR angiography, particularly when the contrast-enhanced methods fail or have serious artifacts.
While long acquisition times of TOF-MRA and PC-MRA have limited their routine use in patients with vascular disease recent research has focused on TSE- or FSE-based approaches (8). ECG-gated FSE-based MRAs are acquired along the axis of the run-off vessels, which allow for larger anatomical coverage in z-axis. FSE-based techniques are typically based on the subtraction of two datasets obtained during systole and diastole with ECG gating. Cine sequences are generally applied to determine the appropriate timing for each acquisition. FSE-based techniques have a high sensitivity and negative predictive value for detection of stenosis of the lower extremities (9). As this approach is based on subtraction of a systolic acquisition from a diastolic acquisition for selective arterial angiograms, it is susceptible to motion artifacts, which may lead to nondiagnostic image quality in up to 50% of patients (9,10). Recent improvements with the development of a variable flip angle with spatially nonselective RF refocusing pulses (SPACE, VISTA, and Cube) can dramatically reduce the data sampling duration because of reduced echo spacing. This technique can also provide more detailed angiography of distal arteries (11).
Until now contrast-enhanced MRA remains the best MRA technique to evaluate arterial disease in the lower limbs, in terms of time effectiveness and accuracy.
TECHNIQUES FOR PERIPHERAL CONTRASTENHANCED MAGNETIC RESONANCE ANGIOGRAPHY
Basics
The basic principle behind CE-MRA is imaging the arterial first pass of paramagnetic contrast agent in the vessels after intravenous injection (12). The injected contrast agent produces a strong shortening of the T1-relaxation time of blood. This results in high signal intensity of the arteries on T1-weighted, spoiled gradient-echo images. The delay between arterial and venous enhancement provides a time window for pure arterial imaging. This time window depends on the rate of contrast agent injection, but also on the anatomic region being imaged. In the upper limbs, venous return is much faster than in the lower limbs, requiring a much shorter measurement time. Since the technique depends on imaging of the first pass of contrast agent, timing the start of image acquisition after injection is essential. Various techniques have been developed to calculate this so-called scan delay, as will be explained later.
The quality of the MRA images with regard to selective arterial visualization, resolution, and volume of interest depends on both sequence parameters used and the contrast
medium bolus geometry. Arterial enhancement depends on individual physiologic parameters, such as cardiac output and blood volume, but also on contrast agent application parameters, which can be manipulated (flow rate, dose, and saline flush volume). The T1-shortening of blood depends on the intravascular concentration of the contrast agent, which, in turn, depends on the rate of injection. In general, the higher the injection rate, the higher the concentration will be, although rates exceeding 5 mL/s do not result in further increase in signal intensity. In fact, intravascular signal may become lower at rates higher than 6 mL/s (13). On the other hand, a higher infusion rate results in faster venous return and a shorter bolus length, requiring a shorter measurement time. Always a compromise between imaging time, resolution and arterial/venous time window must be sought. Since, for examination of the lower limbs, the entire vascular tree from renal arteries to the ankles has to be imaged with high resolution (especially in the lower legs), a long arteriovenous time interval is necessary. Therefore, in the lower extremities injection rates of 0.5 to 1.5 mL/s are used. After injection of contrast agent, the venous system should be flushed with saline to push the contrast medium column from the arm veins into the circulation.
medium bolus geometry. Arterial enhancement depends on individual physiologic parameters, such as cardiac output and blood volume, but also on contrast agent application parameters, which can be manipulated (flow rate, dose, and saline flush volume). The T1-shortening of blood depends on the intravascular concentration of the contrast agent, which, in turn, depends on the rate of injection. In general, the higher the injection rate, the higher the concentration will be, although rates exceeding 5 mL/s do not result in further increase in signal intensity. In fact, intravascular signal may become lower at rates higher than 6 mL/s (13). On the other hand, a higher infusion rate results in faster venous return and a shorter bolus length, requiring a shorter measurement time. Always a compromise between imaging time, resolution and arterial/venous time window must be sought. Since, for examination of the lower limbs, the entire vascular tree from renal arteries to the ankles has to be imaged with high resolution (especially in the lower legs), a long arteriovenous time interval is necessary. Therefore, in the lower extremities injection rates of 0.5 to 1.5 mL/s are used. After injection of contrast agent, the venous system should be flushed with saline to push the contrast medium column from the arm veins into the circulation.
A heavily T1-weighted, spoiled gradient-echo sequence [short repetition time/echo time (TR/TE); flip angle, 25 to 50 degrees] is used in which the achievable field of view, matrix size, scan volume, and number of partitions are determined by the arterial/venous time interval. Subtraction of pre- and postcontrast images is performed to visualize only the arteries for greater ease of image interpretation and to reduce artifacts (Fig. 35.1) (14). The obtained volumetric dataset containing high intensity voxels corresponding to arteries can be postprocessed using the maximum intensity projection (MIP) algorithm. For improved diagnostic performance, review of the individual source images or reformatting images in the transverse plane is required.
Timing of Image Acquisition
In CE-MRA, adequate timing of the start of data acquisition, with arrival of the contrast agent in the vessels of interest, is essential. Arterial enhancement must coincide with acquisition of central k-lines, and for improved arterial/venous differentiation, the central k-lines have to be sampled before venous return. Essentially, three methods have been developed to ensure proper timing.
Test Bolus
Adequate timing can be performed by measuring the circulation time after intravenous injection of a small test bolus (1 to 2 mL) of contrast agent, using a rapid dynamic imaging sequence. With this dynamic series, the arrival time of the test bolus after injection in the region of interest (ROI) can be imaged and, therefore, the scan delay can be calculated. To avoid pooling of the test bolus in veins, the tubing has to be flushed with saline. Both the test bolus and the final infusion should be injected at the same rate. This timing method is fairly robust and easy to perform. A disadvantage is that the method requires additional administration of contrast agent. It lengthens the total procedure time with 2 to 3 minutes. Also, in case of irregular heart action, the circulation time calculated from the test bolus may sometimes differ from that during injection of the final bolus.
Fluoroscopic Triggering
Fast imaging sequences can be used to visualize the influx of contrast into the ROI. MRA data acquisition is then started automatically or manually [MR Smart-Prep (15), bolus track (16)]. A disadvantage of this method is the delay of 2 to 4 seconds that occurs between visualizing the arrival of the contrast bolus and the actual start of acquisition. Also, time for breathing instructions, if required, may be too short.
Time-resolved Imaging
With ongoing improvements in hardware and gradient technology, the time required to image a large volume of interest with high resolution has greatly reduced. With a 2D slab, the entire anteroposterior diameter of the legs can be covered in 2 to 3 seconds (17). Therefore, by performing a dynamic volumetric series after intravenous injection of the contrast agent, the arterial phase will always be included in one of the datasets, thus obviating the need for bolus timing (18).
Imaging Strategies
For imaging of the leg vessels, a large anatomic region (approximately 100 cm) has to be covered. Since the longitudinal field of view of MR systems is limited to 45 to 50 cm, complete imaging of the lower extremity requires imaging of several regions (or “stations”). This means repositioning of the patient to the isocenter of the magnet. Two methods for imaging of the entire legs have been developed: So-called multistation/multi-injection imaging and the bolus chase method. In both techniques one has to ensure sufficient overlap between the separate stations. No studies have been performed comparing the two methods directly; however, because of its short examination time and more efficient use of contrast agent, the bolus-chase technique is the current standard of practice.
Multistation/Multi-injection Imaging or Step-by-Step Technique
With this technique, the three regions of the legs (aortoiliac, femoropopliteal, and infrapopliteal vessels) are imaged sequentially with two or three separate bolus injections of contrast medium (19). Imaging of each station involves acquisition of a mask dataset, which is subtracted from the subsequent images obtained after contrast injection. Contrast agent can be injected at moderately high rates of 1 to 1.5 mL/s, ensuring high signal intensity in the vessels. The advantage of this technique is that it can be applied on any clinical scanner without use of special hardware, and imaging parameters can be tailored to every single station. The multistation/multi-injection technique, however, is not very time efficient (30 to 45 min/patient); a total contrast agent dose of 0.2 to 0.3 mmol/kg/patient is required, and the contrast-to-noise ratio of the subtracted images becomes less at the second and third stations as a result of circulating gadolinium (Fig. 35.2) (20). Using larger dosages in the subsequent stages can partly circumvent this decrease in signal intensity. Surface coils can be used for imaging of the different stations. Huber et al. (21) achieved 100% sensitivity and 94% specificity for identifying 80 hemodynamically significant stenoses and 39 occlusions in 24 patients.
Bolus Chase or Moving-Table Technique
In this technique, mask images of all three stations of the legs are made first (16,22,23 and 24). Then, during slow constant infusion of contrast agent, the flow of contrast through the legs is chased using a floating table. This technique requires application of special hardware to move the table to predefined positions. This can be done using homemade table-stopping devices or special MR-table hardware. The advantage of the moving-table technique is that it is very rapid (coverage of the entire legs in less than 4 minutes), requiring a relatively low dose of contrast agent (approximately 30 cc).
Initially, a disadvantage of the stepping-table technique was that the three stations were imaged sequentially while the scan preparation had been optimized for only a single station. Later on, the method has become more flexible (16), and even dedicated surface coils can be used (25,26).
Although with the bolus-chase technique, imaging times of the various segments are short, early venous enhancement may sometimes cause difficulties in evaluation of the arteries of the lower leg (Fig. 35.3). This venous overlay may be especially prominent in patients with diabetes, venous ulcers, or cellulitis. Enhancement of the veins in the third station, the lower legs, can be minimized by using an optimized imaging protocol: Use of a biphasic injection protocol (e.g., 1 mL/s during 10 seconds and the rest with 0.5 mL/s), use of “centric” k-space acquisition, in which the contrastdetermining k-lines are acquired first, and the use of parallel imaging. With the use of parallel imaging techniques such as simultaneous acquisition of spatial harmonics (SMASH) (27) or sensitivity encoding (SENSE) (28) techniques, combination of coils can be used to compensate for omitted gradient steps. The increase in imaging speed can be used to reduce acquisition times or to increase resolution of the scan (29). Binkert et al. (30) used a dual-bolus technique, in which they started with a dedicated calf MRA and—after a 15-minute interval—proceeded with the moving-table MRA. This led to better visualization of the infrapopliteal arteries with less venous overlay and therefore a significantly increased diagnostic accuracy, compared to moving-table MRA alone. Also, venous compression by means of a tourniquet, either
at the midfemoral (31) or at infragenual level (32,33), can be used to slow down the flow in the vessels of the legs.
at the midfemoral (31) or at infragenual level (32,33), can be used to slow down the flow in the vessels of the legs.
Image Presentation
To encourage acceptance of the technique by referring clinicians, one has to provide them with images to which they are accustomed and which appear similar to conventional angiography. The number of images should be limited as a practical concern for vascular surgeons. The images should be large to be viewed from a distance, displaying standard angiographic views. Also, bony landmarks should be provided for better orientation, similar to images of conventional angiography (Fig. 35.4).
PITFALLS
Although signal loss owing to turbulence and in-plane saturation is usually not present in CE-MRA, overestimation of the length of a stenosis can still occur, especially at high velocity rates at the stenotic area and a low concentration of contrast material (34).
If the contrast agent arrives in the vessel of interest after sampling of the central k-lines as a result of improper timing, a central dark line in the vessel can be seen, the so-called pseudodissection (35).
Care has to be taken to include all vessels of interest in the imaging volume. Inappropriate image coverage may mimic occlusion of tortuous arteries, such as the external iliac arteries (36). Therefore, it is recommended to present also the sagittal or transverse views to demonstrate the entire imaging volume (Fig. 35.5).
COMPUTED TOMOGRAPHY ANGIOGRAPHY OF PERIPHERAL ARTERIES
GENERAL
The introduction of multidetector row or MSCT represented a revolutionary advance in CT technology. Compared with single-slice helical CT, MSCT offers faster rotation times and simultaneous acquisition of multiple helices per rotation, resulting in shorter acquisition times, increased longitudinal volume coverage, lower-dose contrast medium, and improved spatial resolution for assessing smaller arterial branches. With every new generation of multislice scanners, these advantages became more apparent, enabling increased table feed (and therefore shorter examination times) and increased spatial resolution.
Radiation dose is an issue in MSCT, but in the age group of patients with intermittent claudication, it is probably not very important. Moreover, the radiation dose in conventional angiography is approximately four times as high as in MSCT (37).
TECHNIQUE FOR PERIPHERAL COMPUTED TOMOGRAPHY ANGIOGRAPHY
As in CE-MRA, the principle behind peripheral multislice CTA is imaging the arterial phase of contrast enhancement before substantial interstitial or venous enhancement occurs. The advent of multislice scanning has enabled chasing of the contrast bolus while maintaining high spatial resolution.
Three important factors determine the quality of images in CTA (38).
High spatial resolution, especially required for imaging of the infrapopliteal vessels, depends largely on the detector collimation and reconstruction kernel. Although inferior to conventional catheter angiography, CTA can assess
arteries smaller than 1 mm in diameter. Since it is volumetric, CTA allows 3D visualization of the vasculature to separate superimposed structures.
High temporal resolution is achieved from fast gantry rotation. Modern scanners have a gantry rotation time of 280 to 350 milliseconds. Therefore, a volume of data (3 to 4 cm on 64-detector row scanners) can be acquired with full scan mode in less than a half second.
The z-axis coverage per gantry rotation and table speed plays a large role in the CTA acquisition. With 16- or greater detector row systems the tradeoff between acquisition speed and slice thickness is no longer an issue because of larger volume coverage. In fact, 4 cm or greater coverage per gantry rotation from larger detectors introduces the problem of imaging too quickly and thus outrunning the iodinated contrast bolus. Care must be taken to match the helical pitch and table speed with the first circulation of contrast material through the anatomic ROI. The timing of a CTA scan is of critical importance so that the data is acquired during peak enhancement and not before or after the contrast has arrived. Table feed should therefore not exceed 50 mm/s (39).
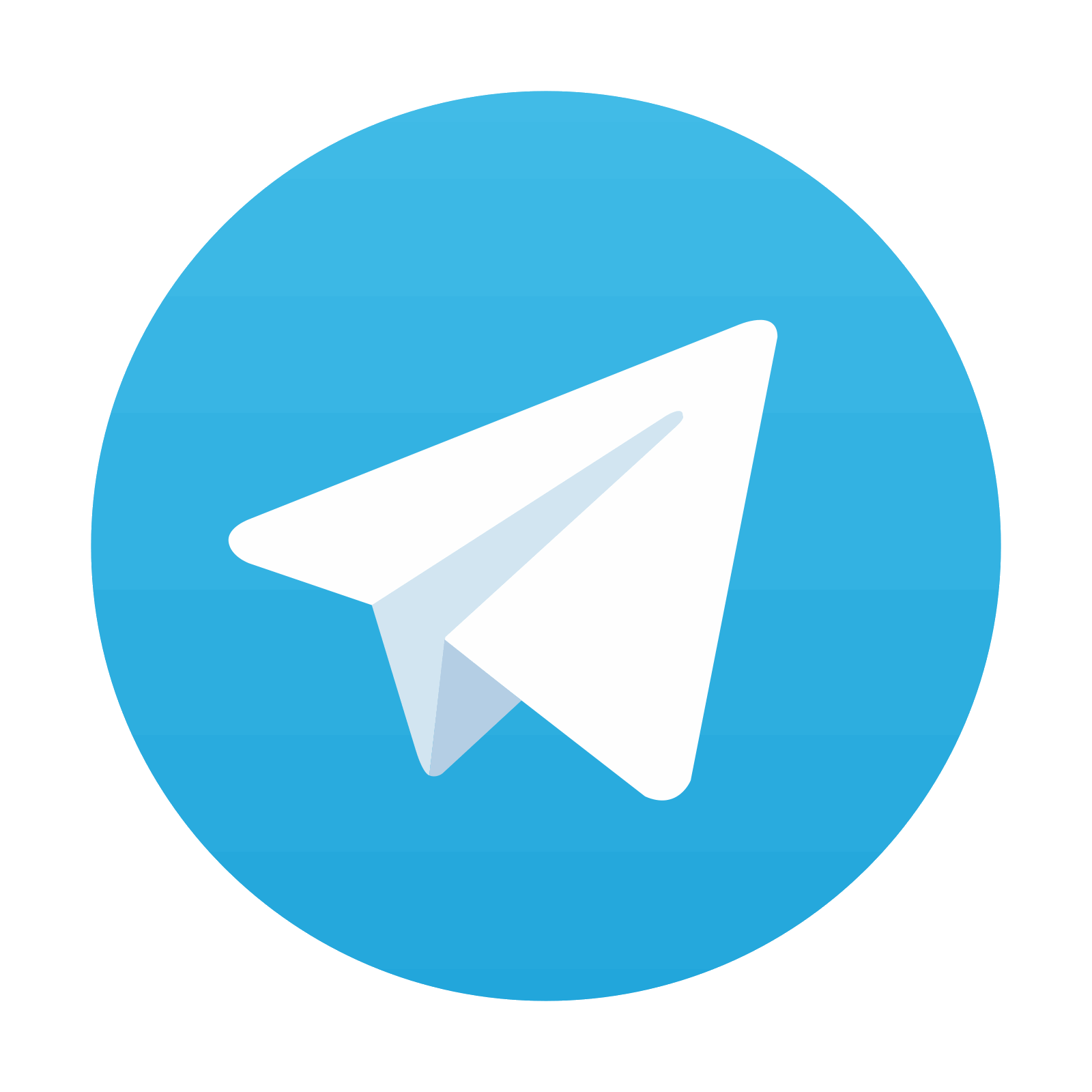
Stay updated, free articles. Join our Telegram channel
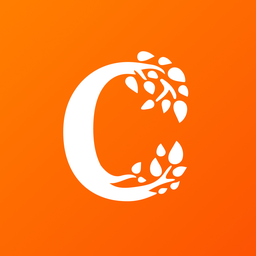
Full access? Get Clinical Tree
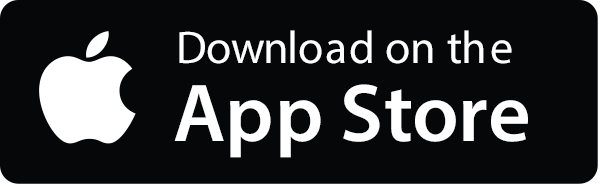
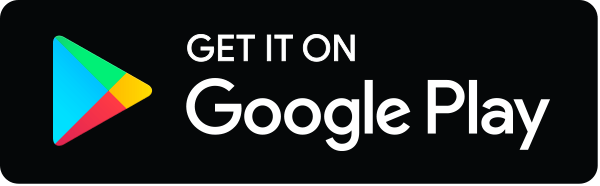